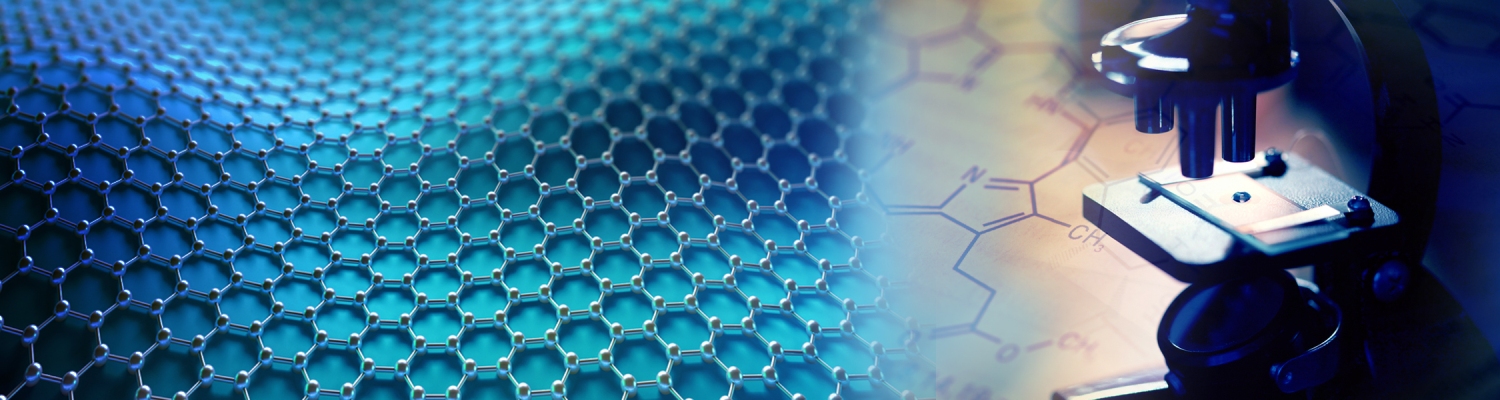
ISSN: 2641-6921
Mohsen Mhadhbi*
Received: February 28, 2021; Published: March 10, 2021
*Corresponding author: Mohsen Mhadhbi, Laboratory of Useful Materials, National Institute of Research and Physicochemical Analysis, Technopole Sidi Thabet 2020 Ariana, Tunisia
DOI: 10.32474/MAMS.2021.03.000174
In this work, a nanostructured TiC powders were produced by mechanical alloying (MA) through high energy ball milling from a mixture of elemental powders of titanium (Ti) and graphite (C) in argon atmosphere. MA process was performed for 20 h in a planetary ball mill (Fritsch Pulverisette 7) at room temperature at a ball-to-powder weight ratio of 70:1. Microstructure and morphology of the samples were investigated by using X-ray diffraction (XRD), laser granulometry, scanning electron microscope (SEM), and transmission electron microscope (TEM). XRD studies revealed that in the times more than 15 h of MA all the raw materials were changed to the desired materials. Results showed that nanocrystalline TiC, with about 10 nm, is synthetized by reaction between Ti and C atoms.
Keywords: Titanium carbide; morphology; nanocrystalline; microstructure; mechanical alloying
Titanium carbide (chemical formula TiC) is extensively used in several industrial applications because of its excellent proprieties, such as high meting temperature (3067 ˚C), elastic modulus, hardness, thermal and electrical conductivity, high wear and abrasion resistance, etc. [1]. Due to these proprieties, it is utilized as cutting tools, abrasives, hard coating to protect the surface of cutting tools from abrasion and wear, aerospace, and others. Moreover, most TiC ceramics are produced according to conventional powder metallurgical processes, which require expansive high temperature equipment. Recently, various new synthetic methods have been used to produce nanocrystalline TiC powders like microwave method [2], thermal plasma [3], sonochemical method [4], mechanochemical method [5], and molten salt method [6]. However, mechanical alloying (MA) is a novel and simple route employed to synthesize materials with a high melting points, which are difficult to be prepared via conventional techniques. It is also a promise technique for producing commercial nanocrystalline powders. It has been revealed that the reaction rate is diffusion controlled, that the diffusion pathway can change during milling process because of lattice strain [7]. It has been concluded that, during MA, the reaction rates are affected by accumulate rate and lattice strain [8].
Several research have been made on the production of nanostructured TiC material from the elemental Ti and C powders. For example, Liu et al. [9] prepared nanosized TiC powders by mechanical alloying of Ti and C elemental powders at various compositions Ti1-xCx (x = 0.35; O.43; 0.50). They found that the grain size of the powders milled for 15 h was in the range of 20-25 nm. El-Eskandarany et al. [10] used a planetary ball mill to synthesize nanocrystalline Ti44C56 powders (with fine cell-like of about 3 nm in diameter) from elemental titanium and graphite powders after 200 h of milling. Tang et al. [11] also fabricated nanostructured Ti50C50 powder (with 6 nm in size) by mechanical alloying from a mixture of Ti and C powder after 80 h of milling. Razavi et al. [12] prepared nanosized TiC powder by high energy ball milling from impure Ti chips and carbon black. After 15 h of milling, the obtained crystallite size was 10 nm. Wu et al. [13] synthesized nanostructured TiC powder by mechanical alloying of a powder mixture of elemental Ti and C. Kudaka et al. [14] prepared nanostructured TiC powder by using a planetary ball mill from powder mixtures of elemental Ti and C with atomic ration 1:1. Xinkun et al. [15] synthesized nanocrystalline TiC powder by mechanical alloying. The average crystallite size of the final product MA for 10 h was about 7 nm. Deidda et al. [16] prepared nanostructured titanium carbide powder by high-energy ball milling through a Spex 8000 Shaker mill under argon atmosphere. Loshe et al. [17] synthesized nanostructured TiC powder via milling using a magneto ball mill under helium atmosphere from initial titanium and carbon powder mixture. Additionally, Mateazzi et Le Caer [18] prepared nanostructured TiC powder, with a particle size in the order of 20 nm, by mechanical alloying from a mixture of titanium and graphite powders. They found that the measured lattice parameter was a = 0.4311±0.0002 nm. Ye et Quan [19] used mechanical alloying to prepare nanostructured compound TiC powder with a grain size of 9 nm after 27 of MA. Yang [20] used horizontal rotary ball mill to prepare nanostructured TiC powder, after 210 min of milling, from elemental titanium and graphite powders.
This study was embarked to evaluate the crystal structure and morphology of the titanium carbide powders before and after mechanical alloying process.
Materials
A mixture of elemental Ti (< 40 μm, 99.9 %, Prolabo) and carbon (5 μm, 99.9 %, Fischer scientific) was sealed into a stainlesssteel vial (45 ml in volume) with 5 stainless steel balls (15 mm in diameter and 14 g in mass) in a glove box filled with purified argon to prevent oxidation. The ball-to-powder mass ratio was 70:1. The mechanical alloying procedure of 20 h was performed by using a high-energy ball mill (Fritsch Pulverisette-7 planetary mill). The milling was interrupted after a selected milling time and powder was collected from the vial.
Characterization methods
The crystalline properties of the obtained powders were characterized by X-ray diffraction (XRD) using a D8 Advance-Bruker diffractometer with Cu Kα radiation (λ = 0.15406 nm) operating at 40 mV and 40 mA. For detailed X-ray line profile analysis, step scan data (step size of 0.015˚ 2θ and counting time f 30 s) of unmilled and milled samples were recorded for the entire angular range 5-80˚ 2θ. The existing phases were determined by the High Score Plus program based on the ICDD PDF2 data base and the diffraction crystallite size was determined by the Full Prof program [21] using the Rietveld method [22]. The particle size distribution and the surface area of the milled powders were determined using a laser analyzer Malvern Mastersizer 2000. The morphology of the samples was studied using a FEI Quanta 200 environmental scanning electron microscopy (SEM) coupled with EDAX. The transmission electron microscopy images were employed for the investigation of the internal nanostructure of the sintered powder. The analysis was completed using a FEI Tecnai G2 high resolution transmission electron microscopy (HRTEM) operated at 200 kV with a spatial resolution of 1.9 Angstrom.
Scanning Electronic Microscopy Study
(Figure 1) shows SEM micrographs and the corresponding energy dispersive X-ray microanalysis (EDX) of the TiC powders before milling and milled for 20 h. For the case of the un-milled powders (Figure 1a), the grains are principally formed by a particles powder with an irregular shape. For an advanced milling time up to 20 h (Figure 1b), the powders having a homogeneous morphology composed by fine agglomerated particles with an average particle size of about 1 μm. This morphology is caused by the repetitive welding, fracturing and rewilding powder particles, which are caused by high energy ball mill. The X-ray microanalysis of the unmilled powders at the point A reveals the presence of the major peaks corresponding to Ti and C elements. After 20 h of milling (microanalysis at the point B), we note the presence of peaks of iron (confirmed by XRD), caused by the wear between the balls and vial surface, and a peak of oxygen due to the surface contamination of the powders before analysis.
Figure 1: SEM micrographs and X-ray microanalysis of the TiC powders (a) un-milled and (b) milled for 20 h.
Study of particle size distribution
(Figure 2) shows average diameters (D50) and particle size distributions of TiC powders milled for different times. The median diameter of TiC un-milled powders is 21 μm. After 3 h of milling, the median diameter decreased to reach 16 μm due to the deformation of titanium particles powder to flake shape causing by the impact of balls during milling process [9]. For an advanced milling time (up to 20 h), we can observe the formation of an aggregate of TiC powders having a diameter of 3 μm. We also note the apparition of two peaks indicates the rod shape of particles powder. The variation of specific surface area, S, of the TiC powders before and after different milling time is illustrated in Table 1. As can be seen, the specific surface area increases with increasing milling time from 0.577 m2/g for the un-milled powders to 3.930 m2/g after 20 h of milling. This great increase can be attributed to the fragmentation of brittle particle powders caused by MA process, which gives the powder high reactivity.
X-ray diffraction analysis
(Figure 3) illustrates XRD patterns of TiC powders milled for different times. As it can be seen from this figure, the diffraction peaks of Ti and C are clearly visible for un-milled powders. For milling time up to 5 h, there was no significant new phase formation and the MA process caused decreasing of the mean crystallite size, increasing of the mean microstrains, and introduces defects. These phenomena lead to a decrease of the intensities of the diffraction peaks of Ti and a broadening of widths. Ren et al. [23] attributed these phenomena to the large number of dislocations that resulted from heavy deformation generated by high-energy ball milling. However, after 5 h of MA, we can be seen that the peaks of carbon vanish completely, which can be attributed to X-ray absorption and possibly to dissolution of carbon in titanium or at grain boundaries and defects in the titanium matrix. Wu et al [9]. revealed that the absence of the carbon diffraction peaks in a MA TiC powders was explained by the location of C atoms at the grain boundaries of Ti grains prior to the combustion reaction [13]. Since 3 h of MA, we can be seen the apparition of a new peak corresponding to the iron element, which resulted from the wear between the balls and vial surfaces during repetitive abrasion and collision. This feature is also indicated by EDAX analysis. After milling for 3 h, the new phase relative to TiC, exhibiting a FCC structure type NaCl with the Fm-3m space group, starts to take place. After 10 h of milling, the formation of TiC was completed illustrated by the disappearance of all the diffraction peaks of Ti in the presence of the broad TiC peaks. Longer milling times (15 and 20 h) led only to the refinement of TiC particles and the introduction of strain. The calculated parameters of the TiC powders after different milling time, obtained by Rietveld method, are summarized in Table 2. As can be seen, the lattice parameter, a, reaches a value of 0.42898 nm after 20 h of milling. This decrease can be attributed to the compressive stresses generated by MA process [24]. Nevertheless, the mean crystallite size of TiC powders decreases rapidly from 49 to 9 nm by increasing the milling time from 30 min to 20 h, while the mean micro strain increases to reaches a maximum value of 0.28 % after 20 h of milling. These changes are related to the fracture of starting powder particles caused by high-energy ball milling.
Transmission electronic microscopy study
(Figure 4) shows the bright-field TEM micrograph and the corresponding selected area diffraction (SAD) pattern of TiC powder milled for 20 h. We can see the presence of fine crystallites having an irregular shape with an average size of about 10 nm, which is in good agreement with those obtained by XRD (9 nm). The SAD pattern consists of discontinuous rings representing the presence of finely grained polycrystallites. It can be indexed as (111), (200), (220), and (311) planes of TiC phase, which are confirmed by XRD measurements.
Figure 4: Bright-field TEM image and corresponding selected area diffraction pattern of TiC powder milled for 20h.
Mechanism of TiC powders during mechanical alloying process
(Figure 5) shows the milling ability of the balls. When the powder particles were milled at high energy by the small balls (as in the case of our work), they are enclosed in a small space ringed by the balls and then, the most particles entered in high frequency collisions uniformly with the nearby balls. This phenomenon is very useful for the mechanical alloying of titanium carbide powders.
Nanocrystalline TiC powders were successfully prepared from Ti and C elemental powders via mechanical alloying for 20 h. Since 5 h of MA, we are obtained a single phase cubic solid solution TiC, which was accompanied by lattice expansion. The average crystallite size reached about 10 nm after 20 h and the average micro strain reached about 0.28 % with a specific surface area of 3.93 m2/g. The raising of milling time leading to a decrease in the crystallite size and an increase of microstrain. With the increase of the milling time, the powders became fine well distributed.
No funding to declare.
The author declares that they have no conflict of interest to this work.
We would like to express our special appreciation and thanks to Ms. Kelly Heidi, Editor Manager in Modern Approaches on Material Science, for her support.
Mohsen Mhadhbi: https://orcid.org/0000-0003-1488-8699.
Bio chemistry
University of Texas Medical Branch, USADepartment of Criminal Justice
Liberty University, USADepartment of Psychiatry
University of Kentucky, USADepartment of Medicine
Gally International Biomedical Research & Consulting LLC, USADepartment of Urbanisation and Agricultural
Montreal university, USAOral & Maxillofacial Pathology
New York University, USAGastroenterology and Hepatology
University of Alabama, UKDepartment of Medicine
Universities of Bradford, UKOncology
Circulogene Theranostics, EnglandRadiation Chemistry
National University of Mexico, USAAnalytical Chemistry
Wentworth Institute of Technology, USAMinimally Invasive Surgery
Mercer University school of Medicine, USAPediatric Dentistry
University of Athens , GreeceThe annual scholar awards from Lupine Publishers honor a selected number Read More...