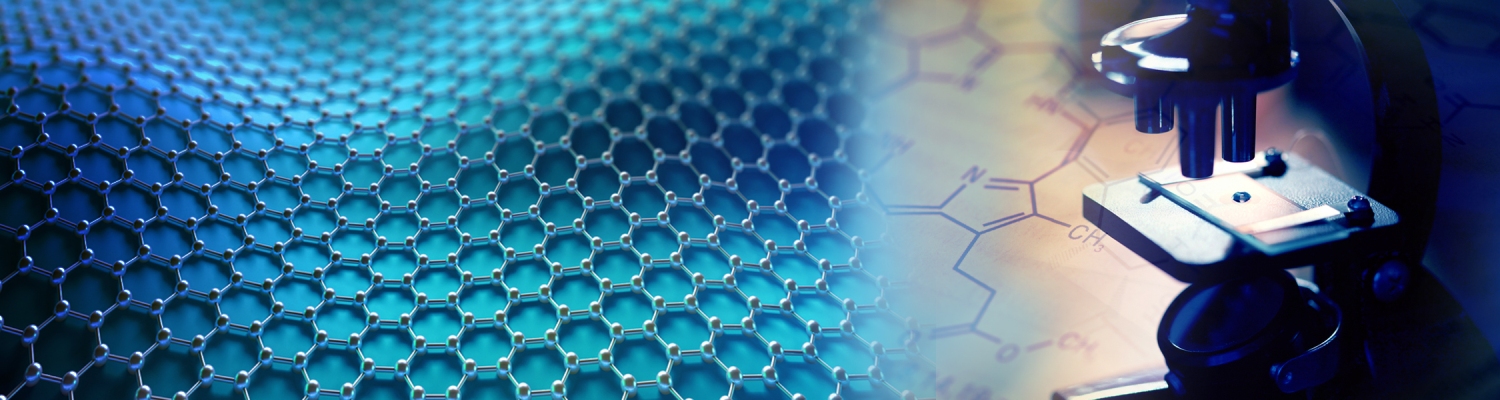
ISSN: 2641-6921
Brenet F, Furgeaud C, Nicolai J*
Received:May 23, 2019; Published: June 04, 2019
*Corresponding author:J Nicolai, Institut Pprime, UPR 3346, Université de Poitiers, France
DOI: 10.32474/MAMS.2019.01.000117
In this paper, Ti3SiC2, Ti3AlC2 and Ti2AlC thin films were synthesized using a two-step method consisting on TiAl2 magnetron sputtering deposition and post-deposited annealing at 800°C or 1000°C. X-ray Diffraction (XRD) and Transmission Electron Microscopy (TEM) were used for the phase identification and microstructure studies. We focused on the kinetic effect of MAX phase growth influenced by annealing time of one and several runs. The results obtained show that for one annealing during 30min we synthesized Ti3AlC2 or Ti3SiC2 respectively for 800°C and 1000°C. Although the total thermal energy provided by annealing steps is equal in each case, one annealing run leads to a very pure 312 MAX phase (Ti3AlC2 at 800°C and Ti3SiC2 at 1000°C), while the interruption during the annealing and the increase cooling step number for the same total annealing time leads to the 211 MAX phase for 800°C and to a mix between Ti3AlC2 and Ti2AlC for 1000°C.
Keywords: MAX phase; Thin films; Epitaxy; Magnetron sputtering; kinetic effect
Mn+1Xn phases (n =1-3) consist in a large class of nanolaminated materials where M is an early transition metal element, A is an A-group element and X is either C or N [1-5]. For n = 1,2,3 the MAX phases are respectively called 211, 312 and 413 due to the periodic arrangement of their structure: n M6X octahedrons separated by a layer of A element in a hexagonal structure. This particular structure gives to the MAX phases a unique combination of metal and ceramic properties, opening the way to a large field of applications [6,7]. The Ti3SiC2 and Ti2AlC MAX phases have been extensively studied due to their excellent properties, including irradiation resistance [8-10], oxidation resistance [11] and electrical properties [12,13]. Several different techniques have been used to synthesize bulk MAX phases. Among these techniques, the most widespread is hot isostatic pressing (HIP) [14] however, various techniques have been developed for the growth of MAX phase thin films using magnetron sputtering technology, either from elemental targets or from compound targets on various substrates [15,16]. As shown on our recent papers, the co-deposition of Ti and Al on SiC-4H substrates leads, depending on annealing conditions, to the formation of either Ti3SiC2 or Ti2AlC [17]. Despite the huge number of studies achieved on MAX phases the formation mechanisms are not well-known. In this paper, we report on correlation between annealing process (temperature, run number, time of run) MAX phase kinetic growth.
SiC-4H substrates, single crystal (0001), n-type, supplied by TANKEBLUE, were used in this study. Al and Ti were co-deposited at room temperature onto SiC substrates by magnetron sputtering using pure Al (99.999%) and Ti (99.995%) targets in a high vacuum chamber (Figure 1), the Ar working pressure has been fixed at 0.3 Pa. Before deposition, the substrate was in-situ cleaned by an etching at 60V during 600s. As shown Figure 1 the fresh TiAl2 deposited layer is nanocrystalline. The samples were annealed in a vacuum lamp furnace (heating rate close to 20°C s-1) at 800°C or 1000°C during one run of 30min or 3 runs of 10 min for each temperature under a pressure around 10-5Pa. Composition and thin film thickness have been checked using X-ray reflectometry (3000 Seifert) and SEM techniques (JEOL 7001 TTLS). The structural investigations were performed by using X-Ray Diffraction (XRD) and Transmission Electron Microscopy (TEM). Diffraction experiments were conducted on a D8 Brucker AXS diffractometer operating in the Bragg-Brentano geometry under atmosphere environment. Diffractometer operates with a Cu tube and the Kβ radiation is absorbed by a Ni filter in order to obtain a pure Cu Kα radiation (λ = 0.15418nm). The LynxEye detector is used for ω-2θ scans with a slight offset to avoid SiC reflections (ω-2θ=0.2°). High- Resolution TEM and Scanning Transmission Electron Microscopy (STEM) images were carried out using a JEOL 2200FS (Schottky- FEG, 200kV). TEM samples were prepared by Focused Ion Beam (FIB) using an FEI-HELIOS dual-beam using the standard lift-out method [18].
Figure 1: a) PUMA chamber scheme. b) TEM micrography of fresh of fresh TiAl2 deposited layer and associated diffraction pattern. The diffraction pattern clearly indicates the nanocrystalline structure of the TiAl2 layer.
Figure 2 shows X-ray diffractograms of TiAl2, 300nm thick on
SiC annealed at 800°C 1 x 30min and 3 x 10min. Both diffractograms
exhibit a peak at 39.2° corresponding to (1014) reflection of the
Ti3AlC2 MAX phase structure. Moreover, the sample annealed
during 1 x 30min shows exclusively Ti3AlC2 MAX phase diffraction
peaks, unlike the 3 x 10min sample also exhibits the (0002) and
(0006) diffraction peaks of the Ti2AlC MAX phase respectively at
9.7° and 39.7°. However, additional peaks could be observed and
attributed to secondary phases. For 1 x 30min, peaks at 42.15°
and 42.71° are attributed to (300) and (112) reflections of Ti5Si3.
For 3 x 10min one peak at 46.0° (not shown here) corresponds to
(200) reflection of tetragonal TiAl2 phase and one peak at 42.7°
corresponds to the (112) reflections of Ti5Si3. The diffraction peak
at 38.9° corresponds to SiC-4H substrate. Figure 3 shows X-ray
diffractograms of TiAl2 300nm thick on SiC annealed at 1000°C for
both annealing strategies. For 1 x 30min, we observed four peaks at
10.05°, 20.12°, 30.37° and 40.82° corresponding to (0002), (0004),
(0006) and (0008) reflections of Ti3SiC2 MAX phase. Moreover, the
only secondary phase presents is the Ti5Si3 structure leading to (300)
and (112) reflections at 42.15° and 42.71°. The diffractogram of 3
x 10min exhibits two peaks at 9.7° and 39.2° corresponding to the
(0002) and (1014) of Ti3AlC2 MAX phase, three peaks at 13.0°, 39.7°
and 40.2° corresponding to the (0002), (1013) and (0006) of Ti2AlC
MAX phase, one peak at 42.1° corresponding to the (112) of Ti5Si3.
Figure 4 shows the thin films obtained on SiC-4H for TiAl2 -300nm
after annealing at 800°C and 1000°C during 30min. The diffraction
patterns obtained and the analysis of HRTEM micrographs reveal
Ti3AlC2 and Ti3SiC2 MAX phase thin film structure consistent with
XRD investigations. Moreover the MAX phases grows in epitaxy
with SiC - 4H following the relation (0001)MAX // (0001)SiC and
SiC. The samples corresponding to 800°C
and 1000°C annealed during 3 x 10min (not shown here) exhibit
the same epitaxial relationship between the substrate and the
layer formed at the interface. However, considering the 1000°C
sample the MAX phase isn’t Ti3SiC2 anymore but Ti3AlC2. STEM
micrography (Figure 5) clearly indicates the presence of two
different structures in the MAX phase layer. Measured spacing
between atomic planes are 1.8nm for the interfacial layer and 1.3
nm for the upper layer respectively corresponding to the 312 and
211 MAX phases. As observed on STEM micrographs, all films,
whatever kind of MAX phases synthesized, are very flat (Figure 6)
and exhibits a very good crystallinity (high chemical homogeneity
and almost monocrystalline). The total thickness of the stacking of
MAX phase are closed to 30nm and 40nm respectively for 800°C–3
x 10min and 1 x 30min and closed to 80nm and 150nm respectively
for 1000°C - 3 x 10min and 1 x 30min.
Figure 2: ω - 2θ XRD diffractograms of TiAl2 - 300nm thick onto 4H-SiC annealed at 800°C during 1x30 min and 3x10 min.
Figure 3: ω - 2θ XRD diffractograms of TiAl2 - 300 nm thick onto 4H-SiC annealed at 1000°C during 1x30 min and 3x10 min.
Figure 4: HRTEM micrography of TiAl2-300nm annealed at a) 800°C – 1 x 30 min and b) 1000°C – 1 x 30 min and associated diffraction patterns. The diffraction patterns can be indexed using SiC-4H and respectively Ti3AlC2, Ti3SiC2 structures on [10- 10] zone axis.
Figure 5: a) HRSTEM micrography of TiAl2 -300nm annealed 1000°C 3x10min, b) and c) represent respectively the Ti2AlC and Ti3AlC2 structure. Black, red and blue dots correspond respectively to C, Ti and Al atoms.
Figure 6: STEM micrographs of TiAl2 - 300nm annealed at 1000°C a) 3x10min and b) 1x30min. The MAX phase stacking layer is respectively 80nm and 150nm thick.
As shown Figures 2,3,4 the annealing of the TiAl2 layer leads to the formation of an epitaxial MAX phase thin film onto SiC - 4H whatever successive runs used (long time or several successive short times). For 1 x 30min the structure of this MAX phase is Ti3AlC2 or Ti3SiC2 depending on annealing temperature, respectively for 800°C and for 1000°C. The formation of 312 MAX phase at low temperature is very interesting and absolutely not expected, indeed, it has been showed that for bulk sample the 312 structure should be formed only at very-high temperature [19,20]. In the case of fast temperature increase, thermodynamical equilibrium isn’t respect anymore. Thus, only thermodynamical consideration used in previous studies aren’t enough anymore and kinetic effect couldn’t be avoid. XRD and TEM experiments indicate that annealing process has an influence on MAX phase structure and properties. Moreover, the MAX phase thickness is also impacted. Indeed, for successive short annealing time, and consequently several cooling temporizations, the layer isn’t as thick as the one long run annealing process sample. For multi-step annealing process we assume that the early stages of MAX phase formation have been already explained as follow:
which is in a good agreement with purely thermodynamic considerations [20]. This kind of reaction leads to an epitaxial growth. Then, the reaction continues with the second step:
leading to the formation of Ti2AlC and extra silicon atoms (Figure 7). We assume that Si segregates above the Ti2AlC and stay at the interface between the MAX phase and TiAl2 layer. Indeed, calculations indicate a fast kinetic diffusion of Al atoms in the TiC structure to form Ti2AlC [21]. Moreover, due to the fact that the Tin+1SiCn system does not have the 211 phase, the silicon cannot substitute Al species in the Ti2AlC MAX phase [22]. During the cooling, the Si reacts with unconsumed TiAl2 to form a very stable Ti5Si3 structure. In the same time, we assume that the upper TiAl2, not yet involved in these two previous steps, will crystallize. Thus, the formation of Ti2AlC MAX phase is a two steps reaction. After cooling, the stacking of all phases is shown (Figure 7), from the top to the interface : polycristalline Ti3Al2 / Ti5Si3 / Ti2AlC / SiC. When the annealing is resumed, Ti element has to diffuse toward SiC interface to create TiC, going through MAX phase or MAX phase and Ti5Si3 either if Ti become from Ti3Al or Ti5Si3. We assume that the stability of MAX phase is enough strong to unconsidered creation of TiC with Ti originated from MAX Phase. By this way the Ti diffusion process is slow, thus the TiC formation is a very slow process. However, it occurs leading to the following reaction (Figure 7):
Figure 7: Representation of the mechanisms involved as a function of the temperature and the number of annealing steps (1x30min or 3x10mn), with τ1 (Ti,Al) and τ²(Ti,Al) represent the diffusion time constant of Ti or Al element from the Fick equations for the one annealing step (1x30min) and several steps (3x10min) respectively.
The described mechanisms occur for both, 800°C and 1000°C, but considering that diffusion mechanisms increase with temperature, we assume that Ti diffusion process is higher for 1000°C leading to a thicker Ti3AlC2 than for 800°C, which is in a good agreement with XRD (Figure 2). Indeed the 312/211 intensity ratio is much higher for 1000°C than for 800°C. Moreover, considering TEM observations, and due to the high theoretical intensity diffraction of (1014) MAX phase plane we considered that the formation following this orientation isn’t really representative of MAX phase growth mechanisms. For one-single annealing process, we consider that the first reactions are similar. Moreover, considering that the thickness of MAX phase formed is thinner for annealing process having cooling temporization, we can traduce this information by a longer diffusion kinetic (longer diffusion time or lengths) of diffusing species. Without cooling, Ti has to diffuse only in TiAl2 and first MAX phase monolayers in growth indeed Nowadays we don’t know if the diffusion of Al is during the cooling or during annealing. If the Al diffuses during cooling only, Ti should diffuse only in TiAl2.Unfortunately, diffusion time and length of Ti in different phases involved is not known. So, the first hypothesis is the increase of Ti diffusion time and length in Ti5Si3 + Ti2AlC than in TiAl2 + Ti2AlC. The second hypothesis is the same concerning Al diffusion kinetics. Thus, the Ti2AlC completely turns into Ti3AlC2. The XRD and TEM experiments show after 1 x 30min that for 800°C the Ti3AlC2 MAX phase is formed while for 1000°C after the MAX phase formed is Ti3SiC2. By this way, we assume that at 1000°C the Si can diffuse through the Ti3AlC2 structure and can substitutes Al atoms of Ti3AlC2 considering the following reaction:
This kind of phenomena has been already observed with Au and Ti3SiC2 [23]. Finally during the cooling the Ti5Si3 structure is formed leading to the stacking: SiC /312 MAX phase / Ti5Si3 / TiAl2 (Figure 7) Representation of the mechanisms involved as a function of the temperature and the number of annealing steps (1x30min or 3x10mn), with τ1(Ti, Al) ) and τ2 (Ti, Al) represent the diffusion time constant of Ti or Al element from the Fick equations for the one annealing step (1x30min) and several steps (3x10min) respectively.
In this paper we demonstrated the possibility to synthesize Ti2AlC, Ti3AlC2 or Ti3AlC2 thin-film onto SiC - 4H using a common two-steps method by tailoring temperature and time running indeed, for similar thermal cumulative energy (30min at setpoint temperature) the obtained MAX phase structure depends on the annealing process. At low temperature (800°C): one single-step process leads to the formation of Ti3AlC2 MAX phase while multistep process leads to the formation of Ti2AlC MAX phase with a few quantity of unepitaxial Ti3AlC2. At high temperature (1000°C): one single step leads to the formation of very pure Ti3AlC2 while multistep process leads to the formation of a Ti3AlC2 - Ti2AlC mixture. We assume that similarly to low temperature the multi-step process decreases the kinetic of the reaction leading to an incomplete transformation of Ti3AlC2- Ti2AlC to Ti3AlC2.
The authors would like to acknowledge H. Bahsoun for the TEM thin foil preparation. This work partially pertains to the French Government program ”Investissements d’Avenir” (LABEX INTERACTIFS, reference ANR-11-LABX-0017-01). This work has been partially supported by ”Nouvelle Aquitaine” Region and by European Structural and Investment Funds (ERDF reference: P-2016-BAFE-94/95).
Bio chemistry
University of Texas Medical Branch, USADepartment of Criminal Justice
Liberty University, USADepartment of Psychiatry
University of Kentucky, USADepartment of Medicine
Gally International Biomedical Research & Consulting LLC, USADepartment of Urbanisation and Agricultural
Montreal university, USAOral & Maxillofacial Pathology
New York University, USAGastroenterology and Hepatology
University of Alabama, UKDepartment of Medicine
Universities of Bradford, UKOncology
Circulogene Theranostics, EnglandRadiation Chemistry
National University of Mexico, USAAnalytical Chemistry
Wentworth Institute of Technology, USAMinimally Invasive Surgery
Mercer University school of Medicine, USAPediatric Dentistry
University of Athens , GreeceThe annual scholar awards from Lupine Publishers honor a selected number Read More...