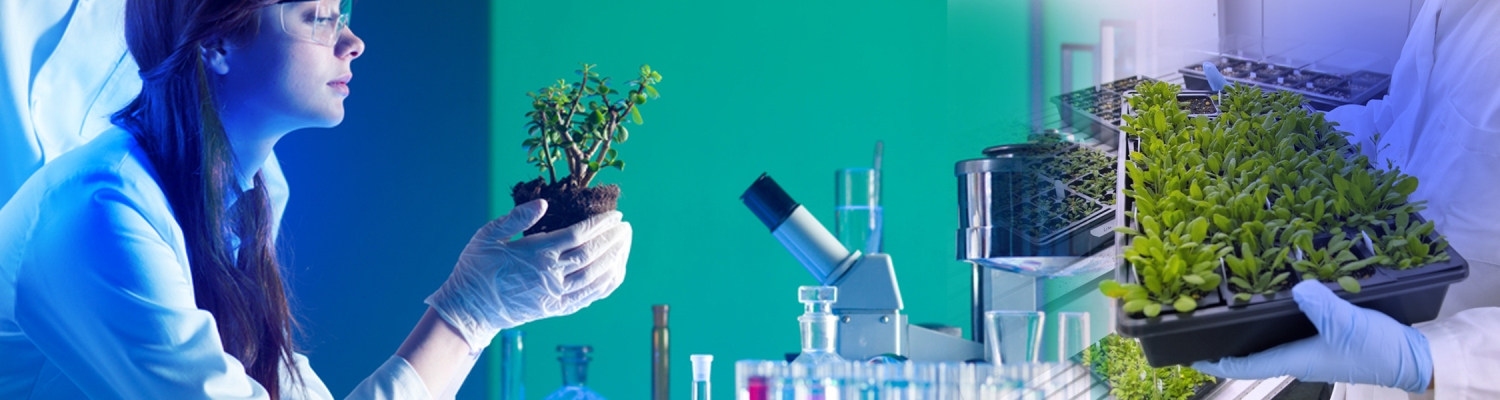
ISSN: 2641-6794
Steven I. Apfelbaum1*, Fugui Wang2 and Ry Thompson2
Received:December 21, 2021 Published: January 11, 2022
Corresponding author:Steven I Apfelbaum, Applied Ecological Institute, 17921 Smith Road, Brodhead, WI, 53520, USA.
DOI: 10.32474/OAJESS.2022.06.000239
We analyzed soils from 30 farms covering ~120,000-acres (48,563 ha) over a studied ~ 7-million-acre (~2.8 million ha) area in the Upper Columbia Plateau region of Washington, Idaho and Oregon, United States. The farms studied used Low Disturbance Cropping (LDC) practices, further defined as one pass no-till farming. In 2012, and again in 2019, we sampled and analyzed soil carbon stocks to a meter depth to understand baseline and short-term changes in soil carbon stocks. We document an average increase in soil organic carbon of ~2.2 tonnes CO2e/ha-year across all sampled fields and a rate of 1.01 tonnes CO2e/ha for higher precipitation upper slopes, and 0.36 on lower slopes in this higher precipitation region. In the lower precipitation areas of the region, upper and lower slope position settings had nearly identical accrual rates of 0.90 and 0.93, respectively. With exception of a few explainable outliers, LDC farm soil organic carbon increased on all farms, and this study suggests this same outcome is possible in the plateau over vast acreages once converted from conventional tillage to LDC crop farming practices. Costs were reduced significantly for landscape-scale sampling by use of high-resolution biophysical stratification, by significantly reducing the number of samples to robustly sample soil carbon stocks over this landscape during the baseline sampling and further reduced upon resampling.
Keywords: Low Disturbance Cropping; Soil Organic Carbon; Regenerative Agriculture; Soil Health; Ecosystem Services
Soil organic carbon is a primary contributor to soil health, defined as soil conditions able to support food and fiber production, and providing and maintaining ecosystem services [1-3]. Soil carbon is a foundational measure that encompasses and supports soil physical, chemical, and biological properties, all important for regulating a healthy soil environment. Improving soil carbon can lead to increased plant productivity, water quality, drought and extreme weather resilience, carbon (C) sequestration, and reductions in greenhouse gas emissions [3,4]. Soil carbon has recently attracted attention, to understand climate mitigation potential and as a proxy indicator of soil health [5,6]. Studies focused on assessing and prescribing cropland fertility, typically sample to soil depths of 15-30 cm. By contrast, this study followed the Verra’s Soil Carbon Quantification Method [7], a standard method for carbon market projects that begins with a biophysical landscape stratification and requires a minimum 1 m depth sampling or resistance to sampling, resulting in a shallower sampling depth being achieved, followed by laboratory combustion analysis, and then requires remeasurement to follow baseline stock changes at the same georeferenced sample points over time. In this study we sought to clarify fundamental questions and often confusion in the soil carbon literature because of sampling to different soil depths and by use of different sampling protocols. Most agronomy cropland sampling is to shallow (e.g., 15 cm) depth and focused on determining crop amendment needs. Some soil carbon studies have used this same shallow depth, and the prevailing published depths range from ~15 cm-1.5 m sampling depths, contributing to significantly different conclusions about soil carbon dynamics, [8-10]. In this study, we have asked the following questions:
a) Can soil carbon stock changes at different soil depths and environmental relations be accurately measured, accounted, and related at the farm and landscape scales?
b) Can the use of high-resolution biophysical stratification drive down sample sizes and reduce soil carbon measurement costs?
c) Can 15-30 cm depth cores predict soil carbon stocks to 1 meter depth?
d) Can farmers adopt Low Disturbance Cropping (LDC) farming to increase carbon stocks on their land as a climate mitigation strategy?
The Palouse agroecological region is a large area of windblown dune-like landform deposits of glacial derived siltsized soil particles (a.k.a. loess) carried varying distances on prevailing westerly winds from the Cascade Mountains, in eastern Washington, western Idaho, and northern Oregon, USA. Windsorted larger and heavier mineral particles deposited closer to eastern foothills of the mountains while finer, lighter particles carried further eastward in the wind. This Palouse study region was selected because of the reasonably uniform geological age, soil minerology, landforms, meteorological zones, and land-use history [9,10]. During the Holocene this region (Figure 1) was dominated by perennial grasslands with deep rooted cool and warm season bunch grasses [11-18]. During periods of erosion and deposition, soil horizon burial and morphogenetic development has occurred through downslope erosion and deposition and under volcanic pumice deposits, including Mt. St Helens May 1980 eruption that blanketed parts of the region with up to a foot or more of ash. The multiple buried horizons and soil morphogenesis cycles are conspicuous in highway road cuts and down cut riverbanks [19]. This study sampled the upper, extant, soil system, avoiding buried historic systems. A 2011 Conservation Innovation Grant (USDA, NRCS) funded the landscape-scale measurement of soil carbon stocks in the Palouse region. Here, we report on the 2012 baseline sampling of biophysically representative ~120,000 acres (48,563 ha) dispersed across this ~7-million-acre sampled area (~2.8 mln ha) of this ~30-million-acre (12,140 mln ha) landscape, using Verra’s Soil Carbon Quantification Method (7) followed by repeated sampling of the same sampling points in 2019. Previously reported soil carbon stocks, accruals, the regional erosion history, and soil carbon ages informed our study (11, 12,13). These regional studies include some of the nation’s longest term agricultural and soil studies (e.g., Pendleton ARS Lab, OR (53, 55), and the Palouse history, vegetation composition, and a well understood archeological and paleontological history (9).
In fall 2011, we pre-sampled soils under different tillage methods, landform positions, and in paired biophysical settings with the same crop rotation: hard or soft winter or spring wheat alternating with a pulse (e.g., lentils, garbanzos) under different tillage practices, including: conventional tillage, chisel plowing (e.g., conservation tillage), 2-3 pass no till, and Low Disturbance Cropping (LDC), one pass no-till. The sampled LDC lands were all member farmers in Shepherd’s Grain, a group of producers of high protein grains using innovative farming practices, including LDC and just in time nitrogen fertilizer delivery. Conservation Reserve Program set aside lands and reference natural areas, including several Palouse prairie remnants, were also sampled. This paper reports the findings from baseline sampling (2012) and repeat sampling (2019) on LDC farmed operations. LDC farming, unlike 2-3 pass and other tillage methods, retains >60 % of the crop residue and disturbs <10% of the soil surface during planting.
Study sites included the primary biophysical conditions and land management history present on regional farms screened by this study team of ecologists, soil scientists, and crop production experts. Primary variables and sampled field land management history were confirmed through review of NRCS crop records and interview with the NRCS soil conservationist and the farmers, then pre-sampling was used to better understand relationships between landforms and the distribution of carbon stocks. The topographically diverse Palouse landforms were mapped and sampled using mapped soils, landform, slope gradients, slope aspects, land cover classifications, soil texture, mineralogy and parent material, and soil profile depth, draped over Digital elevation models for the entire region. Detail about geomorphic position and related soil processes, including surface shape (concavity/convexity), slope position, rates of erosion and deposition, drainage and water regime, historic and existing ecology, and plant community, and cropping practices, land use and management history and duration, and farming history and landscape modifications were factored into the presampling location randomized selection. Using GIS, these data were assembled and the ~7-million-acre area was stratified using high resolution data sets providing a maximum resolution of 1 m2 on-the-ground pixel size.
In LDC farm fields, we initiated this study by conducting presampling to understand the prevailing variables that explained the distribution of carbon stocks on the landscape (50). This focused to understand the distribution of soil carbon stocks across different landform positions in three locations across the Palouse region; seventy-one 1-meter length cores collected across the landform positions and upon analyses we found greater than 90% of the variance in estimating soil carbons stock means were explained by three variables: Slope Aspect (Acode); Slope position (Scode), Precipitation (Pcode), and Cropping/tillage history (Hcode) as defined in Table 7. The overall regional data analysis was rerun with the regional soil carbon sampling that (see below) documented robust relationships between these same variables and the carbon stocks present in the sampled biophysical strata found across the region.
Farms included in this study were screened to ensure the LDC practice had been used for a minimum of 5 years and were Shepherd’s Grain members. In addition, local soil and water conservation district staff and Shepherd’s Grain producers helped to locate non-LDC neighbors practicing conservation and conventional mold-board tillage. We used the pre-sampling results and confirmed comparable understandings based on the regional soil carbon literature (11, 12,13) to develop GIS mapping of these settings to create a final biophysical stratification over a ~ 7-million-acre landscape. Once mapped, we randomly stratified sample points allocated over ~120,000 acres (~48,563 ha) of private farms and public lands we could access, that included the Shepherd’s Grain farms.
Carbon stocks measured from 173 pairs of cores with a length of 80 cm in 2012 and 2019 documents average soil organic carbon stocks significantly increased 15.12-tonnes CO2 equivalent/ha (CO2e) (p<0.0001) between 2012 to 2019, with an increase at least 8.64-tonnes CO2 equivalent/ha at the higher and 0.13 at the lower 95% confidence level, respectively. The average annual increase was 2.16-tonnes CO2e/ha-yr. Carbon stock relations with the environmental factors of PCODE, SCODE, ACODE, and the HCODE “ as “precipitation (PCODE), slope(SCODE), aspect (ACODE), and the history (HCODE) of were fitted using generalized linear mixed model to the measured carbon stocks (Table 1). A best fitted model with lowest AIC and gamma distribution was selected for interpretating results and found carbon stocks were not significantly affected by NE and SW (p=0.54) aspect differences. HCODE alone was also not significant effect on the carbon stocks (p=0.99), but it played an indirectly effect through PCODE (p<0.0001 for PCODE; p=0.046 for interaction effect of PCODE and HCODE). SCODE significantly affected the carbon stock (p<0.0001), but no interaction was found between SCODE and HCODE (p=0.42).
Carbon stocks showed a linear positive relationship from P3 to P5, with the highest stock in P5; lower slopes also had ~22% higher carbon stocks than up slope locations. Carbon stocks among the three levels of H-CODE were not significantly different. Though HCODE affected the carbon stock in different precipitation zones (PCODE), there were no significant differences by HCODE. Carbon stocks in H3 and H4 within P4 zone were not significantly different with any combination of PCODE and HCODE, implying carbon in P4 was in a transition status between P3 and P5, except for H3 in P4, a lower carbon stock (Table 1). Carbon stock change comparisons 2012 and 2019 revealed of the four environmental factors that significant differences were only correlated with precipitation zone (PCODE, p=0.011), and through interaction with Slope position (SCODE, p=0.02); thus, through interaction effects between PCODE and SCODE. Carbon stock changes were not accounted for by differences in SCODE (p=0.53) and HCODE(p=0.43). The highest significant carbon stock changes were measured in upper slopes in P5 (~ 68% higher than the lowest carbon change) in the upper slopes of P3 region. The carbon change in P4 region, regardless of slope, was in a transition zone between the P3 and P4 in both other slope positions (Table 2).
Table 1: Carbon stock at 80 cm depth by environmental factors and conservation agriculture practices.
Table 2: Carbon change at 80 cm depth by environmental factors and conservation agriculture practices.
Carbon stock measured from 264 pairs of cores with length 15 cm in 2012 and 2019 documents average soil organic carbon stock significantly increased 3.95-tonnes CO2 equivalent/ha (CO2e) (p<0.0001) over the 7 years, with an increase at least 2.52-tonnes CO2 equivalent/ha at lower 95% confidence level. The average annual increase within the 15cm depth zone, was 0.36-tonnes CO2e/ha-yr.
Carbon stock relations with the environmental factors of PCODE, SCODE, ACODE, and the HCODE conservation agriculture were fitted using generalized linear mixed model to the measured carbon stocks (Table 3). A best fitted model with lowest AIC and lognormal distribution was selected for interpretating results and found carbon stocks were not significantly affected by NE and SW (p=0.216) aspect differences. HCODE alone was also not significant effect on the carbon stocks (p=0.67), but it had an indirect effect through PCODE (p=<0.0001 for PCODE; p=0.0078 as an interaction effect between PCODE and HCODE). SCODE significantly affected the carbon stock (p=0.0033), but no interaction was found between SCODE and HCODE (p=0.86). Carbon stocks showed a linear positive relationship from P3 to P5, with the highest stock in P5. Carbon stocks among the three levels of H-CODE was almost identical. Though carbon stock by HCODE, as a fixed main factor, was not significant among the three levels; HCODE affected carbon stocks within each PCODE. For instance, within P3, carbon stocks at H5 were significant different from H4 but not H3 (Table 3). Analysis of relationship of carbon change between the two years with the four factors revealed that carbon change was only significantly different by slope aspect, (ACODE, p=0.0336). The carbon change was not significantly different by PCODE (p=0.48), SCODE (p=0.72) and HCODE(p=0.74). No significant interaction among the four factors occurred (Table 4).
Table 3: Carbon stock at 30 cm depth by environmental factors and conservation agriculture practices.
Table 4: Carbon change at depth 30 cm by environmental factors and conservation agriculture practices.
Carbon stock measured from 251 pairs of cores with length of 30 cm in 2012 and 2019 documents average soil organic carbon stock significantly increased 6.12-tonnes CO2 equivalent/ha (CO2e) (p<0.0001) over the 7 years between 2012 to 2019, with an increase at least 3.69-tonnes CO2 equivalent/ha at lower 95% confidence level. The average annual increase was 0.53-tonnes CO2e/ha-yr. Carbon stock relations with the environmental factors of PCODE, SCODE, ACODE, and the HCODE conservation agriculture were fitted using generalized linear mixed model to the measured carbon stocks (Table 5). A best fitted model with lowest AIC and lognormal distribution was selected for interpretating results and found carbon stocks were not significantly affected by NE and SW (p=0.59) aspect differences. HCODE alone was also not significant effect on the carbon stocks (p=0.69), but it played an indirect effect through PCODE (p<0.0001 for PCODE; p=0.012 for interaction effect of PCODE and HCODE). SCODE significantly affected the carbon stock (p=0.0003), but no interaction was found between SCODE and HCODE (p=0.87). Carbon stocks showed a linear positive relationship from P3 to P5, with the highest stock in P5. Carbon stocks among the three levels of H-CODE was almost identical. Though carbon stock by HCODE, as a fixed main factor, was not significantly different among the three levels, HCODE affect with carbon stock occurred within each PCODE . For instance, within P3, carbon stocks at H5 were significantly different from H4 but not H3 (Table 5). Analysis of relationship of carbon change between the two years with the four factors revealed that carbon change was not significantly different by any one of the four factors (Table 6).
Table 5: Carbon stock at 30 cm depth by environmental factors and conservation agriculture practices.
Table 6: Carbon change at depth 30 cm by environmental factors and conservation agriculture practices.
All but five of the sampled farms had measured net positive increases in soil carbon stocks between 2012 to 2019 (Tables 7 & 8; Figure 2). Stock changes at the individual farm-field scale ranged from 0.06 to 7.93 tonnes CO2e/ha-yr. averaging 2.28 tonnes CO2e/ ha over the seven years. Of the five farms, two showed significant reductions in soil carbon stocks of -13.0 to -128.0 tonnes CO2e/ ha. The largest decrease was a farm sampled for the first time in 2019; that did not participate in the 2021 baseline sampling. Thus, the decline resulted because of our mathematical procedure of subtracting the 2012 stock measurement from the 2019 measurement. The -13.0 was an explainable outlier as we learned that the 2012 sampled points coincided with the construction of a new farm roadway where both topsoil stripping and upgradient erosion and deposition over our 2012 sampling points, explained the changes observed in the soil core strata, and the measured carbon stocks. We evaluated all sites through discussion with farmers and NRCS conservation staff and review of NRCS farm records and aerial photograph to ensure that only LDC farming occurred on the sampled farm fields and that accrual rate measurements used in this analysis were accurate. One farm was eliminated from further analysis because they discontinued use the LDC cropping practice within 1-2 years after the baseline sampling was completed in 2012. We also use objective third party 2012 and 2019 soil horizon descriptions from the same sampled points between 2012 and 2019 to further evaluate individual soil sample points and outliers, such as described above were eliminated from further analysis and are shown as zero (Table 8). All remaining sampled points and the measured carbon stocks and accrual rates were accepted for this analysis. Once justified outliers were removed, this resulted in a slight decline in the recalculated carbon stock variance among all farms, and a small annualized average carbon stock increase to 2.28 tonnes CO2e/ha-year, (Tables 7 & 8). Outliers have been converted to an accrual rate of zero in this tabulation.
Table 7: Precipitation zone (Pcode= 18 inches), slope positiom (Scode=upper,mid, lower slopes), slope aspect (Acode=NE vs NW) and Tillage type (H1-H3) are coded in this tabulation.
The following are code definitions used in this analysis:
Pcode (Precipitation Zone), P2=09-12”, P3=12-15”, P4=15- 18”, P5=18-25”, P6=25-54’: Acode (Aspect Zone) = SW vs NW facing; Scode (Slope Position Zone)= Upper, Middle, Lower; Hcode= (Cropping history), Conventional tillage, LDC no till for 0-5,5-12, 12-20, and > 20 yrs.
The 2012 project budget was funded by a USDA Conservation Innovation grant of $1.2 million dollars. This funded the stratification, farm enrollment and contract development and execution to allow access, pre-sampling, and the creation of a sampling plan for each farm. It also funded the actual soil core sampling (mobilization, labor, expenses, and laboratory costs), three educational events for farmers over the region, interviewing each farmer, and drafting, finalization, technical peer review, and approval of the Verra VM0021 protocol. All data analysis and reporting of the soil carbon data, when received from the laboratory, was also funded by the grant. The 2012 budget for stratification, pre-sampling, full region sampling, lab analysis, and reporting was $300,000 and this was applied to 120,000 acres in 2012. In 2019 the same all-in costs were $150,000 and included engagement with farmers to gauge interest in participation in carbon project, repeat sampling at farms of interested LDC farmers, laboratory and data analyses and summary reporting of carbon change (farmer specific summary reports). With minor changes in participating farms, the same LDC farms were sampled in 2012 and 2019 using the stratification and sampling plans developed in 2012. We calculated at a high-level cost comparison between 2012 and 2019, (Table 9).
Table 9: Cost estimates for All-In Costs for deploying VM0021 over ~120,000 acres of 7 million acres of the Palouse Columbia Plateau region.
This cost has eliminated from resampling in 2019 the 2012 sampled representative CRP fields, conventional tilled farms (e.g., mold board plowed fields, chisel plowing, etc.) and also several reference natural areas (e.g., native Palouse grassland remnants and nature preserves). The 2012 sampling of these few sites, during the pre-sampling was undertaken to understand the potential regional controls and statistical bracketing opportunities by measurement of the carbon stocks under these non-LDC land use practices. The costs were not covered by the grant monies. Based on this analysis, because the entire 7 million acres has been stratified and was equally represented in the land-base that could have been sampled, it appears that the cost per ha ranged from $ 0.017 - $0.0021 dollars/ha (0.021 to 0.043 dollars/acre) (USD, 2012). Assuming a carbon value of $16/ tonne CO2e and 0.8 tonnes CO2/acre-yr accrual (=2.08 tonnes CO2e/ha-yr) this suggests a value of $276/ ha ($112 dollars per acre) in carbon value accrued over the seven years between 2012 and 2019. Converted to the cost per acre, this value when multiplied by the average cost per acre for sampling suggests the carbon measurement cost per acre ranged from $1.95 - $0.97 dollars/ ha ($1.95 $2.40 to $4.80 cost) over, 2012 and 2019, respectively.
Re-use of the 2012 stratification reduced by half, the overall cost for 2019 resampling. The stratification produced statistically reliably carbon stock measurements across the large region for the prevailing biophysical strata. The use of the landscape stratification and distribution of randomly sampled points across this landscape saved significant sampling time and added efficiency. For example, compared to random sampling without the biophysical stratification, the efficiency would not have been increased nor costs reduced. The pre-sampling of correlates with the distribution of soil carbon, improved the cost efficiency, also improved the sensitivity to detect changes. The costs are likely to decrease with improved carbon measurement in situ technologies (and that) become available, while project scale increases.
These concerns and the operational costs to simply mobilize to each sample point suggests sampling at each point to collect 1-meter depth samples provides greater understanding and certainty in carbon stock measurements and dynamics. If the same number of subsamples were analyzed for 15, 30, 50 and 80cm core sample lengths, the costs would be identical for all core sampling and lab analyses. However, because we instructed the lab to subsample the 1m length core for soil carbon analysis in four stratum layers (compared to the 3, 2 and one less analysis for the 15,30 and 50 cm core lengths), the additional soil carbon and bulk density measurements in longer cores results in a greater expense. There is additional cost for the additional analysis to the 1m depth, but sampling to this depth adds deminimus operational costs while simultaneously contributing operational efficiency, and data about carbon stock dynamics at depth. This has a soil carbon market-place benefit by also reducing the discounts applied to the resulting modeling uncertainty to predict overall carbon stocks using shallower sampling depths. The outcome of discounting can result in less revenue for participating farmers.
Asked differently, if the mobilization cost to each sample point represents 40% of the sampling cost (including all-in sampling and lab costs), does it make sense to only sample to a 15 or 30 cm depth? This study suggests sampling only to 15 and 30 cm depths (Table 10, Figures 5A & 5B) because of the significant organic carbon stock increases we have measured to nearly one meter depth (see next analyses in this paper) may neglect to document the carbon accrual dynamics. In fact, measuring down from a very dynamic soil surface, sampling shallowly, provides no confidence of repeated sampling actually measuring the same soil stratum because of erosion and deposition dynamics at the surface. And, in non-LDC farm fields there is increased uncertainty because of the changes in surface soil depth and compaction with tillage. Sampling to 1-m provides “situational awareness” by being able to rapidly determine changes, (like we determined in our outlier analyses) in farm operations, erosion, or deposition that would not otherwise be detected with shallow sampling.
Table 10: Soil organic carbon measurements (mean +/- StD) at 15 and 80 cm depths based on baseline sampling in 2012 and follow- up sampling in 2019 at the same sample points.
Figure 3: Soil Carbon Accrual Location Vertical Mapping, by Soil Profile Comparisons 2012 to 2019, Palouse Agroecosystem, Washington, USA.
Figure 4: Soil carbon accrual location and depth mapping, by soil profile comparisons 2012 to 2019, Palouse agroecosystem, Washington, USA.
Figure 5: Soil organic carbon (kg/m2) comparison for the 80 cm core length compared with the 0-30 cm core depths. Abscissa is soil organic carbon stocks in the “A” horizon that averaged 26 cm depth; Ordinate axis is soil organic carbon stocks from 27-80 cm depth.
We also ran the analysis for the full 80 cm core samples using Israel (1992) at a 95% confidence level (p< 0.05) and a 10% margin of error. In 2012, for 1m length samples 42 to 44 soil cores were needed to estimate carbon stock to 15 cm depth, and 53-56 samples were required to an 80 cm 100 cm depth: 9-14 additional samples for the longer cores. The differences in the coefficient of variation between the shallower and longer cores suggest lower variance is achieved with fewer deeper samples. Using Verra’s VM0021 [7], we measured significant soil carbon accruals to 85cm [10], and accrual rates of ~ 2 tonnes CO2e/ha-year, comparable with Palouse LDC rates. As in [10-25], in this paper we also document significant carbon accruals occurring to 80+ cm soil depths (Figures 4A-4D). Assuming accruals to 80 cm, because the cost to mobilize and collect the sample, transport, and process samples are essentially the same for each core length, the increased carbon stock measured over the 80 cm depth (0.65 kg/m2 of C) as opposed to 0.11 kg/m2 in the 15 cm depth cores, suggests that the average increase of 5.9x (590%) in soil carbon stocks sampled at nearly identical sampling costs may offset the additional costs for the increased number of lab analyses over the 80 cm to 1m depth samples. This may suggest sampling to a meter depth after expending the cost to mobilize to sampling points and suggests the 9-14 additional core samples to achieve a 95% confidence level and 10% margin of error in sampling is an important fixed cost appears to contribute disproportional high value to understand carbon stock dynamics and levels.
To evaluate the vertical accrual of soil organic carbon stocks in the soils, for the Palouse, we normalized soil core lengths to 80 cm and created per centimeter of length average soil carbon stock profiles to better understand the differences between soil carbon stocks by precipitation zone, slope position, and slope aspect (Figures 3A-3D). This analysis also suggests an importance of doing deeper sampling for carbon stock measurement and detection of stock changes (Figures 4A-4D). This analysis suggests a bimodal distribution of increased SOC accruals in the upper 30-40 cm, and a depth gap-where accruals may have been near zero or slightly negative, and then an increase deeper in the soil. The gap appeared to be positioned between 30-45 cm depths. This analysis documents increased SOC to depths of 80 cm in the Palouse can occur under LDC farming. A second analysis looked at the predictiveness of using the “average “A” soil horizon (“topsoil depth in 2012 and 2019”) (Figures 5A and 5B) of the 0-26 cm depth (on the abscissa in kg/m2), to predict the total soil organic carbon for the entire core, from 0 to 80 cm (on the ordinate in kg/m2) depth. The linear regression r-square of 20% suggests with this high level of shallow depth sample carbon stock variance that it is not possible to make accurate predictions of 80 cm depth soil carbon stocks. Removal of outliers, because of the very high sample size in this analysis (“n” = 641), resulted in no significant improvement in the predictiveness of soil carbon stock changes to the full 80cm depth by use of the 0-26 cm depth carbon stock measurements.
We then evaluated the predictiveness of using the 0-26 cm depth to predict only the 27-80 cm depth soil organic carbon levels (Table 10; Figures 5A & 5B). By subtracting the 0-26 cm depth SOC from the total carbon and treating both depths as independent estimators of soil carbon stocks, this analysis suggests that the high level of variability in the 0-26 cm depth adds significant variance and again using shallower soil carbon stocks to predict deeper stocks is not reliable or even possible in this region. This analysis suggests that direct measurement of the entire 80 cm depth soil horizon may be the only and best way to obtain reliable carbon stock accounting measurements. If more accurate tracking of accruals at depth is best supported by deeper sampling and achieved at lower incremental costs, and if this adds value to landowner management knowledge and potential revenue returns from ecosystem markets, then sampling to 80-100 cm is an important priority and strategy to use in landscape carbon stock measurements. This project also suggests SOC stock measurement can be accurate, cost effective, and is achievable over large landscapes over time. This analysis suggests for the same primary costs (mobilization, labor, all-in operation costs) and the cost of 2-3 additional soil samples/core that would be laboratory analyzed for an 80 cm to 1-meter length core (over the cost of a 15 or 30 cm core), that the 1-meter core sampling and analysis is significantly more cost effective and yields significantly more carbon understanding.
Improvements in soil organic carbon from LDC farming generates multiple benefits far more valuable than the carbon increases might indicate. Shepherd’s Grain farmers found they more reliably meet the high protein quality requirements of the specialty grain crops they grow for a pastry/pizza dough flour product, by using LDC farming. During their early transition years this was achieved with considerable risk and by adding more costly fertilizers to avoid discounting when they went to market where protein thresholds were not met. Crops that fell well short of the threshold could only be sold into the commodity grain market, not to the higher value specialty market. Practicing LDC farmers achieve the higher protein criteria more easily, even during drought years. LDC (one pass no-till farming) reduces the passes over the field to one during all planting operations. There is only one pass during harvesting as well. The number of passes equates to a significant reduction in petroleum fuel, reduced miles of wear and tear on equipment as compared to a 2-3 pass no-till, or conventional tillage, practice. While speculative, we have been told that LDC farmers typically experience a signification reduction in operating costs [26-32]. They have also been able to downsize the horsepower of their tractors to pull the single pass no-till drill and avoided tillage. This saves on the carried capital costs, operational costs, and maintenance costs.
A combination of higher value, non-commodity grain production, coupled with lower operating costs and comparable or better crop yields, reduces costs, and increases profits. While not reported here, we learned that conventional farms using mold board (and other methods of soil disturbing) tillage had negative carbon stock accrual rates, which was consistent with reports by other researchers in the region [33-40]. These outcomes align using LDC, with increasing carbon stocks on the farm. LDC farms have experienced a measured increase in carbon stocks. Based on resampling, the regional average, LDC farms accrued > 2 tonnes/ CO2e/ha-yr. The predicted accrual rate using the time-series chronoseries and linear regression analysis with only the 2012 carbon stocks measurements predicted this identical accrual rate. For the carbon market analysis, on each farm we used slope positions and meteorological precipitation zones as the primary correlates with soil carbon stock changes. This resulted in the following mean annual soil carbon accrual rates, based on the difference between the 2019 and 2012 carbon stocks measured. We defined four strata with the following mean rates of accrual (Table 11).
Table 11: The high precipitation zone had an average of > 45.7 cm (18 in) of annual precipitation while the low precipitation zone had < 45.8 cm.
We found the carbon measurement and prediction results in this study to align closely with other studies in the region. For example, at the Washington State University and USDA/ARS Cook Research Farm, sampling to a 1.5-meter depth documented nearly identical ranges of soil carbon stocks as we have measured (Huggins and Uberuaga, Undated). Stocks ranged from 54 to 272 tonnes C/ha, or 198-998.24 tonnes CO2e/ha. In the 57-ha study site, the lowest stocks were measured on the driest south and west facing ridge tops and slopes, which is identical to our findings in the drier meteorological zones. In both studies, the highest stock levels were measured in the cooler moister north slopes and in the toe of slopes above drainageways. To address the full accounting needs of a baseline estimate of carbon stocks, but also understand GHG emissions on these baseline projections, regional data on GHG emissions were used [41-47]. For purposes of this analysis, the assumptions included no change from baseline in fertilizer use and no change in GHG emissions from petroleum use because both assumptions are conservative baseline scenarios. Further, datasets created by Huggins found when comparing Low Disturbance Cropping and Tillage Cropping with comparable fertilizer uses (formulations, rates, and timing), that the semi-arid areas of the Palouse, had negligible measurable N2O and Methane emissions annually.
The soil carbon stocks, rates of erosion, and soil carbon accrual rates measured in this project fall in line with similar measurements in test plots and demonstration field studies in limited locations of the Palouse landscape [48-52]. In contrast to other projects that have measured “conservation tillage” (Conservation Cropping) and various “residue management” strategies, this project appears to be one of the first where Low Disturbance Cropping has been measured across the diversity of landscape strata and to an 80 cm to 1 meter soil depth. In this project residues were left in place in the fields and continuous Low Disturbance Cropping practices have been ongoing. Typically, other studies that averaged “landscape” carbon accrual rates under unspecified types and durations of “conservation tillage practices” nationally averaged 0.63 tonnes CO2e ha/yr. with a range across the USA of -0.43 to 1.53 while continuous No-Till averaged 1.26 tonnes CO2e ha/yr. with a range across the USA of -0.43 to 3.62 [15]. The rates of soil carbon accrual under continuous No-Till nearly double those rates measured in studies based on unspecified types and durations of “Conservation Tillage” practices. [13, 53-55] During the new study we sampled various No-Till fields (continuous for 5, 10, and 28 years, and the reversion to following ten continuous years with a three-year Conventional Tillage interlude, followed by an additional 1 year of No-Till), and Conservation Cropping Tillage fields, Conventional Tillage fields, and reference native Palouse prairies. Unfortunately, some regional studies only sampled soil carbon to a 20-centimeter depth, but the relative quantities of soil carbon in this depth range aligned with our findings. They found the native prairie remnants to have the highest carbon stocks (63.7 MtC/ha or 233.77 MtCO2e/ ha); followed by the No-Till for 10 years with the Conventional Tillage interlude (58.4 tonnes C/ha or 214.38 tonnes CO2e/ha), then the No-Till for 4 years (50 tonnes C/ha or 183.5 tonnes CO2e/ ha, and lastly Conventional Tillage for over 100 years with 27.9 tonnes C/ha, or 102.39 tonnes CO2e/ha.). Similarly, switches from Conventional Tillage to No-Till farming nationally in the USA has been found to typically sequester 2 to 4 tonnes of Carbon per acre per year [56-62].
Soil carbon losses from the literature as result of erosion are nearly identical to the measurements and averages predicted but not reported here in this paper from this project under Conventional Tillage (0.5 tonnes C/ha-yr. or 1.8 tonnes CO2e/hayr.). Documented soil carbon erosion loss rates in the Palouse of 50- 70%, have increased SOC variability on the land. “Since late 1800’s moldboard plowing in Palouse has been associated with loss of 25 Tg/ha of SOC” [14], which equates to 0.325 tonnes C/ha-year or 1.19 tonnes CO2e/ha-yr. Similar statistics have been documented globally on soil carbon erosion rates of 0.8-1.2 Pg C yr-1, rates of SOC sequestration through conversion to No-Till farming ranges from 100-1000 kg ha-1 yr-1 [16, 63-70].
We have learned the VERRA VM0021 robust biophysical stratification, can help drive down sample sizes and reduce costs for soil carbon measurements on large landscapes. We debated how to calculate the all-in costs for sampling (mobilization, labor, equipment capitalization, lab fees, insurance, fuel), but this surely can be evaluated again when methods for cost analysis are standardized. We costed using a commonly used method that estimates costs from “agronomic study research sized plots”; and determining the number of soil core samples to estimate the means at the plot scale, multiplied by the cost per core sample, and then escalated proportionately to the scale of a landscape to predict landscape scale sampling costs. Compared to our real costs, this plot scale-amplification approach vastly inflates and generates erroneous, significantly higher costs, as the acreage under investigation becomes larger. We have evidence of the exact opposite by use of the stratification process; when the entire landscape is stratified and each stratum is randomly sampled at 1m depths, the variance decreases based on the coefficient of variance, driving down sampling needs and costs.
The direct use of the stratification in this project-- sampling fields in ~120,000 acres of farmland dispersed across the 7 million acres has contributed to a reduced cost/ha. Sampling landscapes relies on the development and deployment of robust standard methods such as Verra VM0021, to accurately predict soil carbon stock changes. A trusted and defensible estimate of stock changes is essential for understanding the benefits of each farming practice and also can deliver greater understanding to the carbon markets and aligning farmer/rancher success with transparent and objective measurements. Most importantly, accurate data is required to be able to build trusting relationships so that landowners, farmers, and ranchers trust the process of measurement and reporting. This analysis suggests randomized shallow soil carbon sampling is unlikely to provide the robust predictive capability of the deeper soil carbon stock measurements prescribed by the VERRA VM0021 method. Perhaps, though conjecture, it would follow that modeling using carbon stock and accrual rate data from shallow sampling results would not have accurately predicted the results. The elevated coefficient of variation of shallow compared to 80cm-1 m sampling, as measured over the Palouse, in one of the most uniform soil settings suggests that a higher variance and lower predictiveness of stock changes is even more likely in more heterogenous soil settings. Further, the often relatively small number of samples taken, and lack of details to antecedent conditions depending on their prior farming history, erosion conditions, different strata present, among many other independent variables, may further challenge carbon stock estimation accuracy from shallower sampling, regardless of the modeling being used. The significant accruals we have measured over LDC farms over the Palouse landscape to 80 cm depths, may also suggest that shallow sampling is unlikely to gain traction through farmer and rancher participation in carbon programs [71-73].
Our analysis suggests soil carbon stocks estimated using sampling depths of 15 to 30 cm is unlikely to create accurate predictions of carbon stock changes with comparable accuracy as a 1-meter depth samples. This analysis suggests core sample lengths of 15-30 cm are not reliable, and perhaps not useful for measuring changes in carbons stocks in the Palouse. In fact, coefficient of variation analysis to achieve p>0.05 with a 10% error in estimating the mean, suggests the 15 and 30 cm samples have a coefficient of variance of 1.39 to 1.59 which is > 2.3 times the COV from the 80 cm to 1m samples (e.g., 0.51). Considering cost effectiveness per unit of data collected and using the Palouse data for testing, this analysis questions incurring all costs to get to a sample point and then to only collect the shorter core sample lengths. Efficiency and data content would suggest the incremental primary additional cost is the laboratory analytics associated with collecting the longer core sample lengths.
Verra’s VM0021 [7] provided a rigorous method to support this projects field sampling design and deployment process and analyses. This systematic method, followed a progression of conservative data analysis steps, to ensure robustness in the estimates of changes in carbon stocks and carbon accrual rate relations over the intervening years between 2012 and 2019. The importance of measuring soil carbon accrual rates increases over time, and that they may continue for perhaps years into the future, is one more finding suggested by the chronoseries analysis completed in 2012. This analysis found the variance in accruals decreased 5-7 years after conversion to LDC farming, and that longer term continuous LDC farming also has a reduced accrual variability [71]. The increased soil organic carbon accrual rates, narrowing 95% confidence limits around the estimated mean, and that this improvement can continue for years, and may not have a temporal termination timeline, based on the 2012 baseline study of some LDC farmers with a nearly 40-year record of LDC data, is an important realization. We will document these time series relationships in a future paper.
Additionally, by sampling to one meter depth, outlier points could be inspected to understand if the soil carbon changes occurred because of scour or deposition that materially changed the soil carbon levels, rather than actual insitu carbon stock accruals. Because the lower soil strata of the 80cm, to 1-meter length cores are typically seldom affected by scour or deposition at depth, the only changes that occur at depth are accruals including changes by water (dissolution and accrual) of mineralized carbon and other mobilized but dissolved materials, and changes in mineral associated carbon.
This study suggests LDC may be an adoptable farming practice that can successfully contribute to increasing soil carbon stocks in the Palouse ecoregion. At this time, we know of only one reason – capital costs for replacing existing no-till drills with one-pass, no-till drills – is why this practice which significantly reduces soil disruption, soil erosion, and requires lower horsepower tractors with lower operational costs, is not being more broadly adopted by farmers.
Innovative finance strategies will likely be required to help farmers who have heavily capitalized investments in large farming equipment (and often relatively new equipment) to trade up to LDC capable drills. Trade up is often associated with a reduced trade-in value, which may not allow the farmers to be able to afford the transition to improved LDC equipment [74-75]. Based on the coefficient of variation being lowest by sampling full 80 cm -1 m cores compared to shallowing depth sampling in the Palouse to a this suggests that shallower depth samples can drive up the number of samples needed to achieve more accurate soil carbon stock estimates. This discounts the value of the carbon stocks and revenues that may be generated for the landowners by neglecting to measure and account for significant carbon stock increases at depth.
We conclude that the additional information benefits from 1m depth soil core sampling more accurately documents change in carbon stocks and accrual rates. This also appears to align with the interests of the carbon marketplace for accuracy and precision and farmers and investors need for a transparent science, and an accurate understanding of soil carbon dynamics.
We acknowledge and appreciate the help of many organizations and individuals that supported this work; USDA, NRCS’s conservation innovation grant program; farmers with Shepherd Grain, University of Idaho soils analysis laboratory, staff of Applied Ecological Services, Inc, and Native Energy.
No Conflict of Interest.
Bio chemistry
University of Texas Medical Branch, USADepartment of Criminal Justice
Liberty University, USADepartment of Psychiatry
University of Kentucky, USADepartment of Medicine
Gally International Biomedical Research & Consulting LLC, USADepartment of Urbanisation and Agricultural
Montreal university, USAOral & Maxillofacial Pathology
New York University, USAGastroenterology and Hepatology
University of Alabama, UKDepartment of Medicine
Universities of Bradford, UKOncology
Circulogene Theranostics, EnglandRadiation Chemistry
National University of Mexico, USAAnalytical Chemistry
Wentworth Institute of Technology, USAMinimally Invasive Surgery
Mercer University school of Medicine, USAPediatric Dentistry
University of Athens , GreeceThe annual scholar awards from Lupine Publishers honor a selected number Read More...