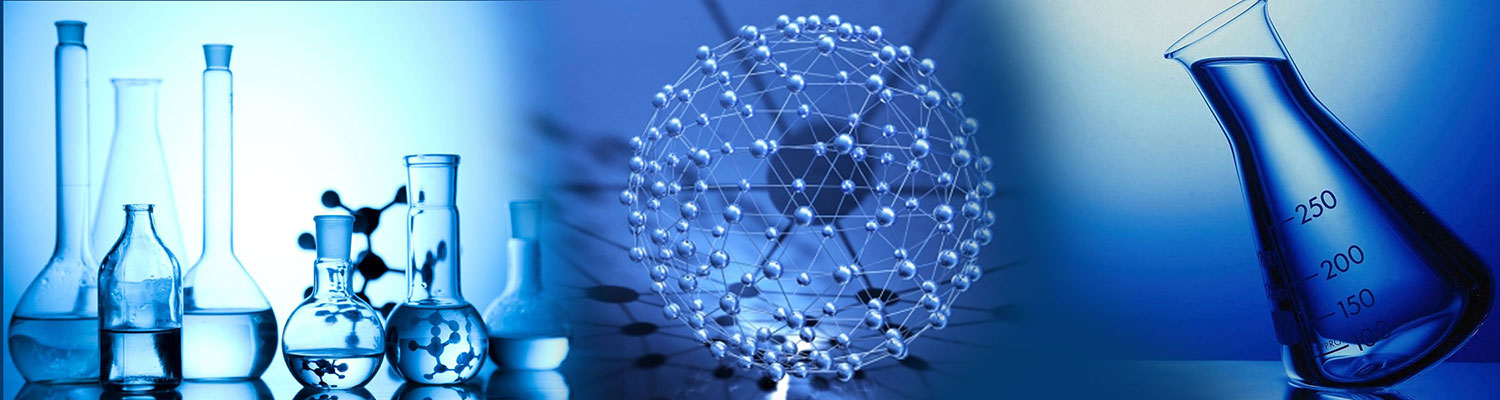
ISSN: 2637-4609
Loai Aljerf1* and Nuha AlMasri2
Received: May 25, 2018; Published: June 11, 2018
*Corresponding author: Loai Aljerf, Department of Basic Sciences, Faculty of Dental Medicine, Damascus University, Damascus, Syria
DOI: 10.32474/AOICS.2018.03.000157
Mercury is extensively used in industry with top usage in electrolytic chlorine. As a result of this elemental consumption in industry, different forms of inorganic and organic mercury get into the environment in great piles every day and many of these mercurial derivatives are converted to methylmercury by microorganisms. The study is assigned to inspect the ecological features of organic mercury species in biological and marine environments. In addition, the paper takes into account the uptake and the distribution of mercury in fish to investigate the conversion and mobilization of mercury from sediment deposits into the general environment. It has been confirmed that the biological half-life of methylmercury in human is about 70 days. In methodology, molecular identification of mercury has been defined. Monomethyl mercury in sediments was analyzed by gas chromatography (GC) hyphenated with electron capture detector (ECD) and the confirmation was measured by mass spectroscopy (MS). The conversion of mercury element to its organic species has been illustrated. In soil, it was found that lower pH favors monomethyl mercury and the higher pH, dimethylmercury formation, respectively. Dimethylmercury is the biological poisoning product and methylmercury is an artifact of isolation procedure. In next paper, we will turn to study the epidemiological features of organic phase of mercury and investigate in deep the distribution, metabolism, and toxicity of mercury and methylmercury in some essential raw food materials, domestic animal feedstock, and some other biological specimens using basically simple analytical methods of chromatography as paper (PC) and thin layer (TLC).
Keywords: Industry, Mercurial derivatives, Microorganisms, Gas chromatography, Methylation rate
The last two decades have dramatized the substantial toxicological significance of particularly methylmercury derivatives. Because the different forms of mercury which get into the environment are usually converted to methylmercury by microorganisms, it is illuminating to examine the sources and applications of mercurial derivatives, both of which are extensive. The world production of mercury in 2017 was estimated by 2500 metric tons [1]. Table 1 illustrates the areas of application, consumption, and percentage of use of mercury in the United States in 2005 [2], wherever, Table 2 lists the types of organic mercuric compounds used in agriculture alone. However, some reports mentioned that the uncontrolled or intentional discharge is believed to account for approximately 5000 tons of mercury per year to the environment [3]. This could be compared with 5000 tons per year of mercury transferred from the continents to the oceans by the rivers following continental weathering. Mercury in fossil fuels can reach values of approximately 0.5ppm [4]. The natural mercury levels in soils and water without industrial or agricultural contamination attains levels of approx. 0.02-0.04ppm for soils and 0.06ppb for water, respectively [5,6].
Air levels of mercury at a particular location are found to depend chiefly on wind direction, wind speed, and seasonal temperature variations [7]. Mercury is introduced into the ecosystem via agricultural uses, waste disposal (mercury used in seals in flow meters, underwater grinders and commutators in waste treatment plants), industrial catalyst effluents, incorporation into products (paints, pharmaceuticals, cosmetics), and accidental misuse as feeding of mercury-treated seeds to farm animals [8]. The adverse effects of mercurial pollution have been extensively reviewed and included:
a) Minamata, Japan [9], where a narcotizing disease of the central nervous system afflicting 3 people of whom 45 died during the period 2000 to 2013.
b) Nigata, Japan, where 26 cases of mercury poisoning and 5 deaths have been documented [10].
The toxicity [11], bio-transformation [12] aspects of adsorption and distribution [13] of organomercurials have been described. The genetic effects of organomercurials include:
a. Mutagenicity of merthiolate in Drosophila melanogaster [14].
b. Mutagenic activity of Mercuran (fungicide containing 2% ethylmercuric chloride and 12% hexachlorocyclohexane) in germinating apple seeds [15].
c. Somatic mutations produced by phenylmercuric hydroxide and phenylmercuric nitrate in flowering plants (seedlings of Raphanus and Zea) and induction of polyploid nuclei.
d. Sticky chromosome and chromosome fragments in root tips of Allium cepa [16]; cytological effects on root cells of Allium cepa of methylmercuric dicyandiamide, methylmercuric hydroxide, phenylmercuric hydroxide and methoxyethylmercuric chloride and the fungicide. Panogen (containing methylmercuric dicyandiamide as the active component) [17].
e. Cytological effects of inorganic, phenyl- and alkylmercuric compounds (e.g., phenylmercuric chloride, butylmercuric chloride and ethylmercuric chloride) on HeLa cell [18].
f. Histological and cytological effects of ethylmercuric phosphate in corn seedlings [19].
g. The C-mitotic action of “Granosan” (fungicide containing ethylmercuric chloride) [20].
h. Agrimax MS4 (containing ethylmercuric chloride and phenylmercuric dinaphthyl methanedisulfonate, respectively).
i. The genetic effects of methylmercuric hydroxide, phenylmercuric acetate and methoxyethylmercuric chloride in Drosophila melanogaster [21] and the induction of chromosome breakage in humans with methylmercury [22]. The complexing and denaturation of DNA by methylmercuric hydroxide has been reported [23]. The teratogenicity of phenylmercuric acetate in mice [24].
j. The embryotoxic effects of “methylmercury” in mice [25].
k. In humans the intrauterine effects of methylmercuric dicyandiamide in Denmark [26] and “methylmercury” in Japan [27] have also been described.
As has been stated earlier, the different forms of mercury from various direct and indirect sources entering into the environment are converted to methylmercury. Wang et al. [28] described this conversion as shown in Scheme 1. Mercury in the first few centimeters (2 cm) of sediment (without microorganisms) is converted to the largest extent to methylmercury. In the next few centimeters in depth of sediment (with microorganisms) the highest rate of mercury methylation is achieved. The methylation rate is correlated with the microbiological activity in the sediment (e.g., higher rate of conversion at higher water temperature and increased amount of nutrients). It was also found that lower pH favors monomethyl mercury and the higher pH, dimethylmercury formation, respectively.
Figure 1 illustrates the conversion of metallic mercury to divalent mercury (Hg0 Hg2+) which has an affinity for organic mud. This binding for organic mud is extremely strong with a coefficient (the measure of the binding strength of a complex) greater than 1021 in comparison to other complexes. This conversion can occur under conditions present at the bottoms of lakes and rivers and has been shown to occur experimentally [29]. The conversion of divalent inorganic mercury to methylmercury (Hg2+ CH3Hg+and CH3HgCH3) has been shown to occur in bottom sediment from aquaria [30], and sediments from a large number of lakes and rivers have revealed microorganisms capable of methylating mercury [31]. The biological half-life of methylmercury in man has been calculated to be about 70 days [32], but its persistence in nature is believed to be much longer. Churchill et al. [33] have estimated that the effects of mercury pollution could last from 10 to 100 years unless control measures are instituted. The biological methylation of mercury in aquatic organisms has been described by Houserova et al. [29] who found that mono- and dimethylmercury (CH3Hg+ and CH3HgCH3) can be produced in bottom sediments and in rotten fish. The same team has attributed the findings to the hazards of mercury pollution in Czech Republic.
The synthesis of methylmercury compounds by extracts of methanogenic bacterium has been described by Wood et al. [34]. The methanogenic organism (MOH) was isolated by Bryant et al. [35] from a symbiotic mixed culture obtained from canal mud at Delft, the Netherlands. Low concentrations of Hg2+ strongly inhibit methane formation, but the formation of B12-r from methyl cobalamin still proceeds and methylmercury and dimethylmercury are found as the sole reaction products by thin-layer chromatography [36,37] or Purge and Trap GC in line with FTIR [38].
Monomethylmercury was analyzed by conventional gas chromatographic [39] detection of CH3HgX (X=halogen) by means of an electron capture detector. Confirmation analyses were performed on an LKB 9000 gas chromatograph-mass spectrometer with the instrument set for detection of m/z (CH3 200 Hg2+); the ionization potential was 20eV. The 0.18 x 180 cm column contained 10% Carbowax 1500 on 60-80 mesh Chromosorb W and was maintained at 150°.
Figure 2 illustrates the concentration of methylmercury in bottom sediment after addition of inorganic mercury followed by incubation for 7 days. Figure 3 shows the concentration of methylmercury in bottom sediment after addition of 10 or 100 ppm of HgCl2 followed by variable times of incubation. The authors’ demonstration of the biological methylation of mercury compounds provides an explanation for the fact that CH3Hg+ is found in fish, even if all the known sources in the environment are in the form of inorganic mercury or phenylmercury and that the formation of the volatile dimethylmercury (bp 94°) may be a factor in the redistribution of mercury from aqueous industrial wastes. CH3Hg+ is soluble in water and is concentrated by living things, usually appearing in body lipids. In part, the concentration may arise by way of the food chain, but fish may also accumulate the toxic ion directly (the concentration factor from water to pike is of the order of 3000 or more). This basic study on the process of methylation appears to be of fundamental significance in the understanding of the uptake and distribution of mercury in fish and the conversion and mobilization of mercury from sediment deposits into the general environment.
Figure 2: Concentration of methyl mercury in bottom sediment after addition of inorganic mercury followed by incubation for 7 days. Lines are drawn between mean values from five samples in two parallel experiment series.
Figure 3: Concentration of methyl mercury in bottom sediment after addition of 10 or 100 ppm of inorganic mercury followed by variable times of incubation. Lines drawn between mean values from five and three samples, respectively.
Concentration of methyl mercury in bottom sediment after addition of 10 or 100 ppm of inorganic mercury followed by variable times of incubation. Lines drawn between mean values from five and three samples, respectively.
After deproteinization, the reaction products were extracted into diethylether, concentrated and subjected to TLC using lowboiling petroleum ether-diethylether (70:30) as developers. Spots were located with 4,4’-bis(dimethylamino)-thiobenzophenone and the RF values of methyl- and dimethylmercury were analogous to those previously reported by Babar and Shinde [36] and satisfied with outcomes of Huang et al. [40]. In order to elaborate whether methylmercury or dimethylmercury was the predominant reaction product, use was made of a general reaction of dialkylmercury compounds with acid, viz., R-Hg-R’+ HCl RH + R’-HgCl. Thus, when hydrochloric acid was added to the standard reaction mixture containing 0.1 μmole of mercury originally as Hg2+, an additional 0.12 μmoles of methane was evolved. (No additional methane was formed in control flasks lacking Hg2+, when acid was added.) Hence, these data indicate that dimethylmercury is the ultimate product of this methyl transfer reaction, although in reactions containing much higher levels of Hg2+, methylmercury is produced. Since acid precipitation of protein [41] is usually performed before the extraction of alkylmercury compounds into organic solvents [42]. It suggested the possibility that dimethylmercury could be the product of biological significance in mercury poisoning, and methylmercury could be an artifact of isolation procedure.
From our experience in this domain, we believe in the possible transfer of methyl groups from Co3+ to mercury in biological systems, may also occur as a non-enzymatic process. Hence, when methyl cobalamin and propyl cobalamin were allowed to react with two individual samples of Hg2+ under mild reducing conditions (Zn dust plus ammonium chloride), the products of these reactions can be identified by TLC as methyl-, dimethyl, propyl-, and dipropyl mercury. The finding of apparent methyl transfer from Co3+ to Hg2+ in biological systems that may also occur as a non-enzymatic system has apparent significance from ecological considerations. Thus, if this methyl transfer reaction is significant in biological systems, then it could be enhanced by anaerobic conditions and by increasing numbers of bacteria capable of synthesizing alkyl cobalamins [43-45]. The authors suggest that pollution of a body of water with nutrients (sewage) will increase the rate of formation of methylmercury at a certain concentration of Hg2+. Methylmercury could be formed by both enzymatic and non-enzymatic reactions, hence making this cumulative poison available for incorporation into various organisms in the aquatic environment and secondarily terrestrial predators.
The action of a mercury-resistant strain of Pseudomonas on organic mercuricmercurials has been reported by Mortazavi and co-workers [46]. This organism was found capable of decomposing phenylmercuric acetate (PMA) into metallic mercury and benzene; ethylmercuric phosphate (EMP) into metallic mercury and ethane; and methylmercuric chloride (MMC) into metallic mercury and methanol [47]. More recently Mahbub et al. [48] described the decomposition of the organic mercurials, phenylmercuric acetate, ethylmercuric phosphate, and methylmercuric chloride by a cell-free extract of the same mercury-resistant Pseudomonas. In the current work, the cell-free extract was freshly prepared by ammonium sulfate fractionation of crude extract obtained from mechanically disrupted cells, treatment at pH 5, and then dialysis. Benzene, ethane and methane were identified by GLC as the products from the decomposition of PMA, EMP and MMC, respectively (Table 3). The decomposition of PMA required the cellfree extract, glucose, NAD or NADP and thioglycolate (at an optimum pH of approx. 6). L-Cysteine, DL-homocysteine, reduced glutathione and mercaptoethanol could be substituted for thioglycolate. The decomposition of PMA also required thioglycolate in excess of its amount to form mercaptide in combination with PMA, and seemed to occur in conjunction with glucose dihydrogenase catalyzing the formation of reduced NAD or NADP. The decomposition of MMC by the cell-free extract was found to occur under analogous conditions found for PMA. The study meets with Tezuka and Tonomura [49] who used the cells of the K-62 strain of Pseudomonas aerobically incubated with 203Hg-labeled or [14C] phenyl-labeled PMA and indicated that about 70% of radioactive mercury or 80% of radioactive carbon disappeared from each medium in 2 h with the concomitant formation of metallic mercury and benzene as shown by GLC (Figure 4).
Figure 4: Gas chromatograph of products derived from phenyl mercuric acetate (PMA) by bacterial decomposition. The organism was incubated with PMA in a 100 ml Erlenmeyer flask with a rubber stopper on a shaker at 30°. After 6 h, 0.5 ml of the gas layer in the flask was removed, and applied to gas chromatography by the use of a Shimazu GC-ZC type apparatus equipped with a hydrogen flame ionization detector (FID). The column used was stainless steel (3 cm x 225 cm) packed with PEG 1000 (A), Thermol 3 (B) or Apiezon L (C).
Table 3: Detection of products derived from decomposition of phenylmercuric acetate, ethylmercuric phosphate and methylmercuric chloride.
Figure 5: Gas chromatograms of products derived from ethylmercuric phosphate (EMP) and methyl mercuric chloride (MMC) by bacterial decomposition. The column used was stainless steel (3 cm x 150 cm) packed with silica gel. (A) Decomposed product of EMP; (B) Decomposed product of MMC; (C) Authentic reagents: (1) Methane, (2) Ethane, (3) Ethylene, (4) Propane and butane.
The organism was incubated with PMA in a 100 ml Erlenmeyer flask with a rubber stopper on a shaker at 30°. After 6h, 0.5 ml of the gas layer in the flask was removed, and applied to gas chromatography by the use of a Shimazu GC-ZC type apparatus equipped with a hydrogen flame ionization detector (FID). The column used was stainless steel (3cm x 225cm) packed with PEG 1000 (A), Thermol 3 (B) or Apiezon L (C). In additional experiments with ethylmercuric phosphate and methylmercuric chloride, metallic mercury, ethane, and methane, respectively, were found as a result of analogous bacterial decomposition (Figure 5).
A hypothetical scheme for the decomposition of phenylmercuric acetate via cleavage of the mercury-carbon bond by a cell-free extract of a mercury-resistant strain of Pseudomonas was suggested by Tezuka and Tonomura [49] as illustrated in Figure 6. It is of interest to note that the vaporization of 203Hg-labeled mercuric chloride by cell-free extracts of drug-resistant Escherichia coli required NADPH and a magnesium ion for maximal vaporization of 203Hg while NADH had only a slight stimulation effect [50]. Since NADPH rather than NADP ions appears essential for the reaction, reduction of mercuric chloride comes out to be necessary for the vaporization of mercury. Cell-free extracts from the sensitive strain have not any vaporizing activity of 203Hg. Chasanah et al. [51] have also reported the reduction of mercuric chloride by mercuryresistant bacteria isolated from air. Ghosh et al. [52] studied the volatilization of mercury from various biological media (e.g., tissue homogenates, infusion broth, plasma and urine) containing mercury as 203HgC12 and found Pseudomonas aeruginosa, Protens spp., and two more unidentified microorganisms present in the water supply that could convert mercuric ion to elemental mercury.
Figure 6: Hypothetical scheme proposed for the decomposition of phenylmercuric acetate by cell free extract of a mercury resistant strain of Pseudomonas (adapted from Tezuka and Tonomura [49]).
Water pollution with nutrients (sewage) increases the formation of methyl mercury. CH3Hg+ is found in fish, even if all the known sources in the environment are in the form of inorganic mercury or phenylmercury and that the formation of the volatile may be a factor in the redistribution of mercury from aqueous industrial wastes.
Bio chemistry
University of Texas Medical Branch, USADepartment of Criminal Justice
Liberty University, USADepartment of Psychiatry
University of Kentucky, USADepartment of Medicine
Gally International Biomedical Research & Consulting LLC, USADepartment of Urbanisation and Agricultural
Montreal university, USAOral & Maxillofacial Pathology
New York University, USAGastroenterology and Hepatology
University of Alabama, UKDepartment of Medicine
Universities of Bradford, UKOncology
Circulogene Theranostics, EnglandRadiation Chemistry
National University of Mexico, USAAnalytical Chemistry
Wentworth Institute of Technology, USAMinimally Invasive Surgery
Mercer University school of Medicine, USAPediatric Dentistry
University of Athens , GreeceThe annual scholar awards from Lupine Publishers honor a selected number Read More...