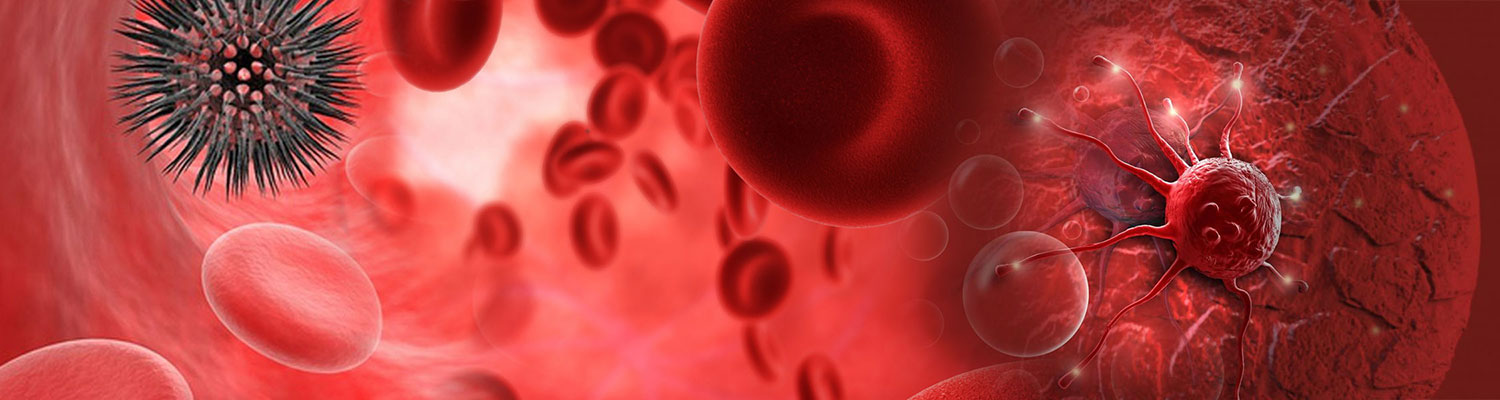
ISSN: 2638-5945
Liebiao Peng1†,Yiqian Wang2† , Rongfei Wang3, and Ming Shao4*
Received:June 14, 2021 Published: July 23, 2021
Corresponding author: Ming Shao, Department of Neurology, Foshan Hospital of Traditional Chinese Medicine, Foshan, 528000, China † These authors have contributed equally to this work.
DOI: 10.32474/OAJOM.2021.04.000196
Objective: To investigate the long-term effect of bone marrow mesenchymal stem cells (BMSCs) transplantation on the recovery of neurological functions following acute cerebral infarction.
Methods: BMSCs were isolated and purified, which were then cultured in neural- or osteoblast-induction medium. Rat cerebral infarction models were established by middle cerebral artery occlusion (MCAO). All rats were randomly divided into 2 groups: the non-transplantation group, and the BMSC-transplant group. In the BMSC-transplantation group, 3×106 or 6×106 Hoechst 33385-labeled BMSCs were injected by tail vein. Nerve Injury Severity Scores (NSS) were used to evaluate the neurological functions after transplantation. Animals were sacrificed at 1, 2, or 3 weeks after transplantation. Histological analysis for Nissl bodies and TTC staining, as well as immunohistochemistry (IHC) staining for GFAP, Ki-67 and NgR were performed.
Results: NSS scores in MSCs-transplantation group were significantly lower in the BMSC-transplant group, as compared with nontransplant group (P<0.01). The cells in the peri-lesional area had more Nissl bodies and Ki67+ cells with BMSC administration, compared to the control group. IHC results showed that the expression of NgR was significantly decreased following BMSC transplantation.
Conclusions: Taken together, our findings indicate that BMSC transplantation can recover neurological functions by promoting cell survival, increasing proliferation, as well as reducing the level of NgR expression in the brain tissue after MCAO.
Keywords: Acute Cerebral Infarction; NgR; BMSC; Neurological Function
Cerebral infarction (CI) represents one of the most common type of stroke, which is attributed to occlusion of large intracranial arteries due to a loss of oxygen to the brain [1, 2]. In addition, neurological damage caused by CI is slow to recover. Current treatment strategies for CI patients are mainly aimed towards inhibiting platelet aggregation and promoting cerebral blood flow [3]. However, the outcomes remain unsatisfactory. Therefore, improved treatment regimens are urgently required. Bone marrowderived mesenchymal stem cells (BMSCs) are a heterogeneous population of plastic-adherent cells known to promote neurological recovery in rats with cerebral infarction, but the mechanisms remain unclear [2, 4]. Many studies have revealed that the failure of neurite regeneration in the early stage of CI is due to the overexpression of myelin inhibitors, including Nogo-A, myelin associated glycoprotein (MAG) and oligodendrocyte myelin glycoprotein (OMgp) which signal through a common receptor complex consisting of the Nogo receptor (NgR), LINGO-1, and p75ntr [5, 6]. Remarkably, Rho A from the GTPase family is a prominent myelin-derived inhibitor of axonal outgrowth [7]. One of the key downstream effectors of RhoA is Rho kinase (ROCK). When activated, ROCK signalling causes the phosphorylation of several downstream targets, regulating cell survival, apoptosis, as well as endocytic trafficking [8, 9]. Upon binding to NgR, Nogo-A activates RhoA-ROCK signalling, which further leads to neurodegeneration in the central nervous system (CNS) [10]. Therefore, targeting Nogo-A has the potential to promote neurite outgrowth and recovery after CI injury.
Recent studies have shown that BMSCs may promote functional recovery of various CNS diseases [11]. Using animal models with CI, BMSC transplantation greatly decreased apoptosis and increased survival of nerve cell [12, 13]. More importantly, BMSCs can produce neurotrophic or growth factors to induce revascularization and enhance the repair of injured cells [14, 15]. A recent study using an in vitro model of spinal cord injury (SCI) revealed that BMSC transplantation significantly enhanced spinal motor neurite outgrowth over Nogo-A repelled spinal neuronal adhesion [16]. In an earlier study using retired breeder rats with stroke, BMSC transplantation led to a reduction of axonal loss and an increase of synaptophysin expression, underlying the therapeutic effects on ischemic brain tissue mediated by BMSCs [17]. However, it remains unclear how BMSCs provide therapeutic benefits in treating CI. In the present study, using a rat model with CI, we aim to investigate the therapeutic effects of BMSC transplantation on neural functional recovery and NgR expression in the brain tissue, especially the longterm effect, which could provide new insights into the treatment for CI patients.
Animal study
A total of 92 SPF Sprague Dawley (SD) rats weighing from 250 to 300 g were provided by the Guangdong Experimental Animal Center (Certificate No. SCXK (Ao)2008-0002) and housed in the SPF laboratory in the Zhongshan School of Medicine of Sun Yat- Sen University (certificate No. SYXK (ao) 2007-0081). This study was approved by the Animal Protection Committee of Sun Yat-Sen University.
Isolation, culture and identification of BMSCs
After rats were sacrificed, femurs were collected, and the femoral bone marrow cavity was washed repeatedly with DMEM/ F12. The cells were centrifuged and resuspended in DMEM/F12 with 10% fetal bovine serum (FBS). All cells were grown at 37 °C and 5% CO2. After 72 h of incubation, the medium was changed and replaced every 2 or 3 days thereafter. When cells reached 80% confluence, they were subcultured at a ratio of 1:3 with trypsin. BMSCs at passage 3 were used as indicated for all experiments. For identification of BMSCs, the cells were incubated with FITC conjugated anti-CD29 and anti-CD34, as well as PE conjugated anti- CD13. At least 1 x 105 cells were acquired and analyzed by flow cytometry.
MCAO rat model preparation
The middle cerebral artery occlusion (MCAO) was developed using a modified method as previously described [18, 19]. Briefly, the rats were anesthetized with 1% pentobarbital sodium (50 mg/ kg body weight) via intraperitoneal injection, the right middle cerebral artery (MCA) was isolated and electrocoagulated for occlusion. After surgery, rats were returned to standard housing conditions.
BMSC labelling and transplantation
1 day after the creation of the MCAO model, approximately 3×106 BMSCs were digested and resuspended in serum-free DMEM/ F12. Then Hoechst 33258 was added to make a final concentration of 10 μg/L. After being incubated for 15 min in a CO2 incubator, cells were washed 5 times in PBS to wash away unincorporated dye. Finally, 3×106 or 6×106 BMSCs were resuspended in 1 ml PBS, and injected into the rats via the tail vein.
Neurological function evaluation
The neurological functions were assessed by neurological severity score (NSS) at 1, 2, and 3 weeks after transplantation with a score range of 0-18. The NSS includes motor coordination, sensory, reflex tasks, and balance tests. Higher scores indicated severer neurological dysfunction.
Histological and immunohistochemical assessment
Rats were anesthetized by intraperitoneal injection with 1% pentobarbital sodium (50 mg/kg body weight) and decapitated. Then, the brains of the rats in each group were harvested, kept at -20°C for 20 min, and cut into 3-μm-thick frozen sections. Hoechstlabelled BMSCs were detected by immunofluorescence staining.
For TTC staining, the brain sections were covered with 1% TTC and incubated at 37°C for 30 min after which the sections were fixed with 4% paraformaldehyde for 24h before analysis. For Alizarin red staining, the cultured cells were fixed with 95% ethanol for 30 min and stained with Alizarin Red Solution for 30 min at 37°C before analysis.
For immunohistochemistry analysis, brain sections were placed into paraformaldehyde (10%) overnight and were then embedded into paraffin. Paraffin sections were cut at a thickness of 3 μm. Antibody against NgR was diluted (1:400, Chemicon) in blocking buffer with biotin overnight, followed by biotinylated anti-rat secondary antibody in blocking buffer for 30 minutes at 37°C. Staining was visualized by DAB substrate kit. Anti-GFAP (Chemicon) was used at a dilution of 1:600 overnight followed by anti-rat/rabbit IgG polyclonal antibody (Dako), and DAB staining. Anti-NF and anti-NSE (Abcam) were used at a dilution of 1:100 for 60 minutes at 37°C, followed by anti-rat/rabbit IgG polyclonal antibody (Dako), and DAB staining.
Statistical analysis
SPSS13.0 was used for statistical analysis. Data were expressed as the mean ± standard deviation (SD). One-way analysis of variance (ANOVA) was used for comparisons among multiple experimental conditions. The student’s t-test was used for comparison between two groups. P<0.05 was considered statistically significant.
Characterization of BMSCs
Primary MSCs isolated from the BM were cultured in flasks. At the initial stage, the cells displayed different morphologies under the microscope. The medium was replaced every 2 to 3 days. On culture days 7 and 8, the cell shape became uniform, and the long fusiform cells were the major cells (80% to 90%). After that, the cells were sub-cultured once every 3 to 4 days. By the 3rd passage, most of the cells were long, spindle-shaped and grow adherently, showing a high nucleus-plasma ratio. By the 15th passage, chrysanthemumshaped clusters of cells were observed (Figure 1A). In addition, flow cytometry analysis revealed that the mesenchymal cell markers CD29 and CD13 were highly expressed, but the cells were negative for the hematopoietic cell marker CD34 (Figure. 1B). To examine the differentiation potential of the isolated BMSCs, passage 3 (P3) of BMSCs were divided into 2 groups: neural cell-induced group and osteoblast-induced group. 1h after being cultured in neural cell induction medium, the cells were small, round or oval in shape. After 5 h, cells had a homogenous, bipolar or multipolar morphology similar to neural cells (Figure 1C). In addition, cells were positive for both neuron-specific enolase (NSE) and neurofilament (NF), which were specific biomarkers of neurons (Figure 1D-E). By analysing cells cultured in osteoblast induction medium, we found that the monolayer became full after 4-5 days substantial morphological change. The cells aggregated and grew in multiple layers without contact inhibition. 3 weeks later, mineralized nodes were found using alizarin red staining under inverted microscope (Figure 1F). Therefore, our results indicate that the cultured cells showed typical BMSC-like morphology and immunophenotype, as well as multi-directional differentiation capability.
Evaluation of infarct damage after MCAO
At 1 day after MCAO, the rats were euthanized, and the brain tissues were stained with 1% TTC after sectioned. Infarcted tissues stained white while normal brain tissues stained red (Figure1G). Hematoxylin and eosin (H&E) staining showed that there was a cribriform reticular change in the necrotic area of right cerebral cortex with nerve cell necrosis, pyknosis of nucleus, disappearance of the nucleolus, and shrinkage and deformation of cell body. The ipsilateral striatum appeared to be pale and loose. In addition, no abnormalities were found in the left cerebral cortex and striatum (Figure 1H). Thus, observations measured by both TTC and H&E confirmed the histological damages and a structural lesion by 24 h after MCAO.
BMSCs improved neurological function after acute in vivo
To test whether transplanted BMSCs can successfully home to the ischemic brain in MCAO rats, we stained BMSCs with Hoechst 33258. After 1 week of transplantation, we were able to detect Hoechst-labelled BMSCs in both the ischemic brain (data not shown) and peri-lesion brain region (Figure 2A). To determine whether BMSC transplantation improved recovery of neurological function, NSS test was performed 1,7,14, and 21 days after MCAO. 1 day after MCAO, rats from both non-transplant and BMSC-transplant groups showed similar neurological function. At 1, 2, and 3 weeks after MCAO, the difference between rats transplanted with 3 x 106 and 6 x 106 BMSCs was not statistically significant. However, the NSS scores from either the two BMSC-transplant groups were significantly lower as compared from the non-transplant group, respectively (Figure 2B). Furthermore, the tail-lift experiment showed that the left forelimb could not be fully erected 3 weeks post-MCAO in the non-transplant group, whereas the left forelimb was fully erected after 2 weeks of MCAO in rats from BMSC-transplanted group (Figure 2C). Our data suggest that BMSCs significantly reduced the neurological function impairment caused by MCAO. To assess the effect of MCAO on neuronal survival, Nissl staining was performed. Neurons with round and palely stained nuclei would be considered as surviving cells, whereas shrunken cells with pyknotic nuclei were considered as dying cells under the light microscope. The cells from all the three groups showed a trend with an increase in the number of nissl bodies in a time-dependent manner. However, compared to the non-transplant group, rats injected with either 3 x 103 or 6 x 106 BMSCs have a significant higher number of nissl bodies at the each timepoint (Figure 2D-E). Our work indicates that BMSC transplantation promotes the survival of neuronal cells induced by cerebral infarction.
To examine the effect of BMSC transplantation on neuronal proliferation, Ki67 staining was performed in the subventricular zone (SVZ) or the peripheral infarction site at 3 weeks after transplantation. We found that the percentage of Ki-67-positive cells in both of the two BMSC-transplant group was significantly higher than non-transplant group. In addition, rats transplanted with 6 x 106 BMSCs had more Ki67-positive cells than those with 3 x 106 BMSCs (Figure 2F-G). The data indicated that BMSC could promote functional neurological recovery partially through promoting cell proliferation.
To determine if BMSC treatment modified the neuroinflammatory response associated with ischemia, we investigated the activation of astrocytes. The analysis of glial fibrillary acidic protein (GFAP) staining in brain sections at 1, 2, and 3 weeks after transplantation found no significant differences between any groups, indicating there was no obvious astroglial mesenchymal phenotype switching following BMSC transplantation (Supplemental Figure S1).
BMSC led to downregulation of NgR expression following MCAO
As the above results show, BMSCs are able to enhance neurological recovery in different aspects. To further investigate the mechanism, we analyzed the expression of NgR in the primary left motor cortex at 1, 2, and 3 weeks after BMSC transplantation using IHC. We found that the levels of NgR showed a decreasing trend in a time-dependent manner from both non-transplant and BMSCtransplant groups. Furthermore, the expression of NgR in 6×106 MSC-transplant group was statistically lower that in 3×106 MSCtransplant group (Figure 3.A-B). Similar results were identified in the primary and secondary right motor cortex, as well as insular cortex, showing that the NgR expression tends to be lower in BMSCtransplant rats, as compared to the non-transplant rats. (Figure 3.CH). Together, our results suggest that BMSC transplantation plays an important role in reducing neurological deficits by downregulating NgR expression.
Figure 1: Identification of BMSCs and examination of the brain tissue damage of in MCAO rats. (A) P3 BMSCs were long, spindle-shaped cells, and P15 BMSCs exerted a shape of chrysanthemum in cluster (x100). (B) Flow cytometry showed that BMSCs expressed CD29 and CD13, while negative for CD34. (C-E) Under microscope, BMSCs adopted a morphology similar to neural cells in neural cell induction medium (x200), as well as positive for NSE (x200) and NF (x400). (F) Alizarin red staining identified mineralized nodes in osteoblast induction medium under inverted microscope (x100). (G) Cerebral infarction detected by TTC staining (n=5). (H) Necrosis was seen in the right cerebral cortex with pale and loose appearance of the ipsilateral striatum. In the contrast, the left cerebral cortex and striatum appear to be intact (x400).
Figure 2: BMSCs improved neurological function by promoting the survival and proliferation of neurons in brain of rats after acute CI. (A) 1 week after transplantation, BMSCs labeled with Hoechst were found in the peri-lesional brain region (x100). (B) NSS results from BMSC-transplant group and non-transplant group at different timepoints as indicated. (C) Comparison of the left forelimb activity between BMSC-transplant and non-transplant groups (n=12 each group). *P<0.05 vs non-transplant group, n=12 rats per group. (D) Representative Nissl-stained images of brain neurons in the peripheral region (x200). (E) Graphs showing the relative number of nissl bodies in infarction site and contralateral site from each treatment group at the indicated timepoints. *P<0.05 vs non-transplant group, n=36 rats per group. (F) Representative images showing staining for Ki- 67 in the subventricular zone (SVZ) (x100) and peripheral infarction site (x100) at 3 weeks. (G) Graph showing the percentage of Ki-67+ cells from each treatment group in the SVZ (n=12 rats per group) and peripheral infarction site (n=36 rats per group) at the indicated time point. *P<0.05 vs non-transplant group.
Figure 4: BMSC administration decreased NgR level in brain tissues in MCAO rats. (A,C,E,G) Representative IHC staining images for NgR in the primary left motor cortex, the primary and secondary right motor cortex, as well as insular cortex at 1, 2, and 3 weeks in each group (x400). (B,D,G,H) Graph showing the percentage of NgR+ cells from each treatment group in the left motor cortex. *P<0.05 vs non-transplant group, n=12 rats per group..
In this study, we investigated the long-term response following BMSC transplantation using an in vivo CI model. We investigated the neuroprotective activities in different treatment groups after 1, 2, and 3 weeks after BMSC transplant. Firstly, we demonstrated that BMSC promoted the functional recovery of neurons. Next, we showed that BMSC prevented the death and promoted proliferation of neurons. Furthermore, we observed that the expression of NgR was downregulated in brain tissue from BMSC-transplant group, as compared to the non-transplant group. These results suggest that BMSCs can promote the restoration of neurological dysfunctions caused by MCAO. It has been assumed that the ability of BMSC to recover neuronal functions is through the secretion of various neurotrophic factors (NTF) such as nerve growth factor, vascular endothelia growth factor and basic fibroblast growth factor [20, 21]. As a prominent myelin-derived inhibitor of axonal outgrowth, Nogo-A has been shown to inhibit the axonal and myelin membranes in different central nervous system (CNS) diseases [22-24]. In our current study, MSCs downregulated the expression of NgR, the receptor for Nogo-A, around the ischemic focus even at 3 weeks after transplantation, suggesting a long-lasting effect on neurological functional recovery. A previous study reported that the therapeutic effects on ischemic brain tissue after BMSC administration lasted at least for 1 year [17]. Further studies using adult rats with chronic stroke confirmed that anti-Nogo-A immunotherapy restored neuronal function when brain damage has occurred [25, 26]. Therefore, future studies should focus on combining the Nogo-A/NgR inhibitor with traditional drug to achieve a better treatment outcome for CI patients.
Matrix metalloproteinases (MMPs) are zinc-dependent endopeptidases that are able to cleave protein components of the extracellular matrix (EM), as well as involved in signal transduction pathways modulating neurite outgrowth and navigation [27]. As previously described, bone marrow-derive MSCs were found to highly express MMPs, which is associated with myelin inhibition [28-30]. There is evidence that MMP-2 is required for the optimal axon outgrowth in mouse retinal ganglion cells [31]. Therefore, to further elucidate the mechanism of downregulated NgR expression by BMSC treatment, an important direction of future research will be to examine the possible interaction between NgR expression and MMPs in the context of CI. In summary, we demonstrated that BMSC administration exerted therapeutic effects on infarcted brain tissue based on a long-term study. More specifically, BMSCs promoted cell proliferation and survival in the peri-lesional brain region, as well as reduced the level of NgR expression. Further research studies may need to elucidate the exact molecular mechanisms, which may lead to the development of therapeutic strategies for acute CI patients.
L.P., R.W., and Y.W. performed experiments and analyzed the data; L.P., Y.W., and M.S. wrote the manuscript. All authors reviewed the manuscript in its final form.
The authors have no conflicts of interest to declare.
The data that support the findings of this study are available from the corresponding author upon reasonable request.
Bio chemistry
University of Texas Medical Branch, USADepartment of Criminal Justice
Liberty University, USADepartment of Psychiatry
University of Kentucky, USADepartment of Medicine
Gally International Biomedical Research & Consulting LLC, USADepartment of Urbanisation and Agricultural
Montreal university, USAOral & Maxillofacial Pathology
New York University, USAGastroenterology and Hepatology
University of Alabama, UKDepartment of Medicine
Universities of Bradford, UKOncology
Circulogene Theranostics, EnglandRadiation Chemistry
National University of Mexico, USAAnalytical Chemistry
Wentworth Institute of Technology, USAMinimally Invasive Surgery
Mercer University school of Medicine, USAPediatric Dentistry
University of Athens , GreeceThe annual scholar awards from Lupine Publishers honor a selected number Read More...