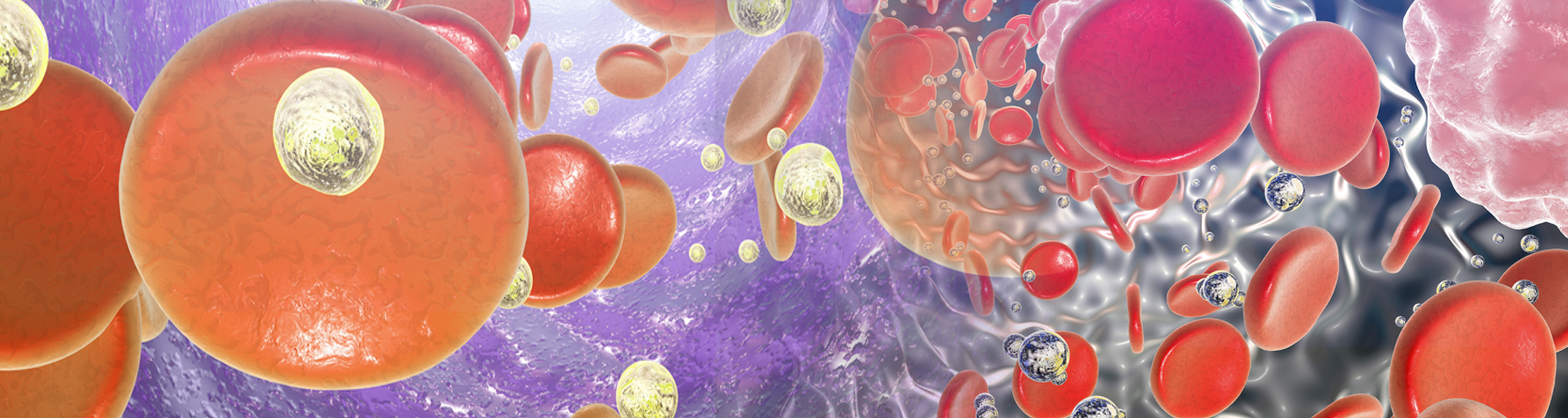
Barbara Roda1,2, Andrea Zattoni1,2, Valentina Marassi1,2, Pierluigi Reschiglian1,2 and Aldo Roda1,2*
Received:October 28, 2023; Published: November 02, 2023
Corresponding author: Aldo Roda, Department of Chemistry “G. Ciamician”, Alma Mater Studiorum, University of Bologna. INBB, National Institute of Biostructure and Biosystems, Rome.
DOI: 10.32474/JBRS.2023.02.000144
Nowadays, the diagnostic challenge requires early, rapid, and cost-effective detection of biomarkers usually present in biological samples at low concentrations. Biosensor devices are effective analytical tools to address these needs. In recent years, biosensors have been proposed to diagnose a wide range of clinical conditions, from cancer diseases to infections and other pathologies [1-5]. Remarkable progress in biosensor technology is consistently achieved through the development of new selective molecular recognition elements, including antibodies, molecularly imprinted polymers, aptamers, DNA and PNA. New highly sensitive, reagent-less transduction principles within electrochemistry, luminescence and more recently plasmonic resonance and Surface Enhanced Raman Spectroscopy have greatly improved the analytical performance of biosensing by simplifying many of the steps required in previous tests. An increased number of biomarkers has been detected across different fields, including clinical, medical, biological, environmental, and industrial sectors, with increased diagnostic and prognostic power [6]. In addition to the use of innovative systems, nanomaterials with tunable properties can be exploited to increase sensitivity in the transducer step [7]. Examples of nanomaterials include stable gold and silver nanoparticles, carbon nanotubes with large surface area and quantum dots used for their color tunability based on their size. In the field of glucose monitoring, metal nanoparticles have been used as nanozymes, colorimetric probes, and in the development of non-invasive, wearable tools [8,9].
The main competition among the different technologies has been related to their limit of detection (LoD), with the goal of achieving single molecule detection at the yoctomolar level. The LoD and limit of quantification (LoQ) are usually determined under ideal conditions to show the potential of a given biosensor. However, these conditions are quite far from the intended use in a realistic setting and cannot guarantee success on a real sample. The equation for the LoD looks simply: LoD = 3σ/S, where σ is the standard deviation of the signal and S is the method sensitivity. However, optimization is a much more complex task, since it involves the (nano)materials recognition element (e.g., a new aptamer, fluorophore, or antibody), or a new sensing scheme using amplified response (e.g., secondary assays, enzymatic amplification). This is where researchers in the field devote the most effort. However, performing analyses in a real scenario while ensuring the laboratory estimated LoD still remains an unrealistic goal without the validation of a given biosensor by properly evaluating its accuracy and response in a real matrix. Most of the published papers and new biosensor formats are far from a real useful application. The authors show the new format still as a proof of concept, with very few applications on real samples, thus limiting the real diagnostic use of new biomarkers usually present at low concentration in biological fluids [10]. Validation with other independent method including high resolution liquid c hromatography-mass spectrometry is not reported in many papers dealing with biosensors. This represents a main drawback and limitation of new biosensors format and advanced technology potentially applicable to real matrix.
The integration of all the steps of the analytical process, including sample preparation and/or clean-up, in a miniaturized format represents the real solution to make biosensors a real product for highly sensitive diagnostic applications. This also applies point-of-care (POC) formats, which can provide additional benefits such as reducing sample volumes, shortening analysis times, and decreasing cost to enhance the potential of personalized medicine. Most of the commercial POCs are related to paper-based and lateral- flow immunoassay format applied to different biological matrices (blood, plasma, urine, and saliva) for semiquantitative results. In recent years, many healthcare companies have distributed products based on biosensors also integrating nanostructured elements for the determination of various biomarkers. Examples include: i-STAT System-blood analyzer assay cartridges for multiparameter determination (e.g., lactate, blood gases, electrolytes, cardiac markers) and Glucocard sensor strips for measuring blood glucose based on electrochemical detection [11,12].
The development of dual detection systems has also made it possible to design devices that provide increasingly accurate and reliable analyses. These devices typically implement colorimetric and optical detection based on the use of nanostructures, manual systems for reagents and pumping, and integrate the biosensor system with rapid and inexpensive detection obtained with a smartphone [13]. Successful use of a biosensor for bioanalysis often requires a preanalytical step to concentrate the analyte and remove potential interferents through sample cleanup. This limits the utility of the biosensors, especially regarding miniaturization and their use as a POC outside a standard laboratory setting. Furthermore, although biosensing systems have improved sensitivity and accuracy, the need for a multi-step process can complicate the use of devices requiring additional laboratory procedures. ample pretreatment thus remains a critical issue since it affects specificity, sensibility, and multiplexed determination of biomarkers in complex biological matrices. Miniaturized systems based on different technologies, including micro solid-phase extraction, magneticassisted bead manipulation, and microfluidic devices have been designed to allow for the direct analysis of biological samples. Among these approaches, microfluidics represents an ideal tool for the rapid direct analysis of biological matrices with an efficient and easy integration with biosensing systems. Several strategies have been devised to enable the loading of low volumes of intact biological samples on the analytical device for further biochemical and diagnostic analysis. These methods use microfluidic systems exploiting inertial effects, microcavities, curvilinear geometries and Di electrophoresis forces.
Some devices implementing microchannels chips acting as micromixers and separative tools were designed for the microbiology and hematological analysis and the screening of blood diseases, the detection of rare circulating tumor and cancer stem cells for an early-stage and specific tumor diagnosis, and the analysis of biochemical parameters [14,15]. The new concept of blood on a chip employing CD microfluidics platform is a rapid and low-cost approach for pretreating whole blood and extract cell-free plasma [16,17]. Integrated microfluidic devices have been described also for the rapid separation of plasma from whole blood samples and the detection of extracellular vesicles and protein analysis for liquid biopsy [18]. Hydrodynamic and dielectrophoretic approaches were combined in a microfluidic channel to separate plasma from fresh blood and allow for real-time optical monitoring of plasma composition. The proposed system uses 2 μL of fresh whole blood and achieves a plasma purity of 99% in just 7 minutes [19]. Sample preconcentration on a microfluidic platform can be achieved through electrokinetic means, filtration, or chromatographic interactions for the ultrasensitive biomarker’s detection [20].
Although microfluidic systems have developed rapidly and extensively, few commercial products are available for the direct analysis of intact biological fluids. The main challenges involve achieving high sensitivity, addressing speciation which may require the separation of single or conjugated analytes, and conducting multiplexed analysis for robust and accurate diagnosis. In the field of microfluidic separation techniques, fieldflow fractionation (FFF) has shown promising for the application to intact biological samples and the integration with specific biosensing tools, with the development of bioanalytical systems suitable for multiplexed analysis of biomarkers. The coupling of FFF with chemiluminescence detection has been proposed for flowassisted, multianalyte assays in heterogeneous phase, and for the detection of intact pathogens on biological samples in an enzyme immunoassay format [21]. FFF was also used for the analysis of plasma proteins and lipoproteins, and for isolation of extracellular vesicles from the plasmas and urines of cancer patients [22-25]. The miniaturized hollow-fiber flow FFF (HF5) performs size separation of intact analytes with low dilution, allowing for the analysis of biological particles with noteworthy benefits: reduced channel volume and operation flowrates, and the potential for disposable usage. HF5 has been proposed as a microfluidic tool integrated into an analytical platform for the fractionation of intact proteins from biological fluids and for proteomic analysis of complex biological samples. It was also used to fractionate and isolate low-abundant plasma proteins and study their interactions with small molecules. Additionally, HF5 was applied to isolate exosome subtypes for a comprehensive particle characterization [26-28].
Further development is required to improve these systems, especially with the advancements in micro/nanofabrication techniques. The advent of rapid prototyping has made it possible to design versatile microfluidics that can serve multiple functions, paving the way for their translation from research to validated clinical applications. Unfortunately, despite the substantial number of published papers dealing with new biosensors designs and concepts, few devices are already commercially available and used at the point of need. Large-scale production of microfluidic-based products is limited by complex design and manufacturing processes that are difficult to industrialize and standardize. The fact that the diagnostic market needs these devices to reduce personnel costs and facilitate diagnosis further confirms that the biosensors are not robust enough and not market ready. We believe that reproducibility and accuracy are still problems and quantitative detection down to a few molecules is still a critical issue, even though the active research activity in bioelectronics and molecular biology seems to fulfill the above criteria in the near future. In addition to the integration of versatile separation and nanotechnology systems into the biosensors, a machine learning (ML) approach could represent a solution for optimizing and simplifying the final design and fabrication of custom devices, which is one of the major obstacles in the development of a fully automated microfluidic platform [29,30]. ML can serve as a control system for all parameters of the analytical procedure, thus minimizing the variability of the entire process and increasing reproducibility and accuracy of the analytical data obtained. Furthermore, by collecting a very large number of data and implementing complex statistical models, the matrix effect can be minimized, allowing ultrasensitive detection of biomarkers even in complex biological samples.
Bio chemistry
University of Texas Medical Branch, USADepartment of Criminal Justice
Liberty University, USADepartment of Psychiatry
University of Kentucky, USADepartment of Medicine
Gally International Biomedical Research & Consulting LLC, USADepartment of Urbanisation and Agricultural
Montreal university, USAOral & Maxillofacial Pathology
New York University, USAGastroenterology and Hepatology
University of Alabama, UKDepartment of Medicine
Universities of Bradford, UKOncology
Circulogene Theranostics, EnglandRadiation Chemistry
National University of Mexico, USAAnalytical Chemistry
Wentworth Institute of Technology, USAMinimally Invasive Surgery
Mercer University school of Medicine, USAPediatric Dentistry
University of Athens , GreeceThe annual scholar awards from Lupine Publishers honor a selected number Read More...