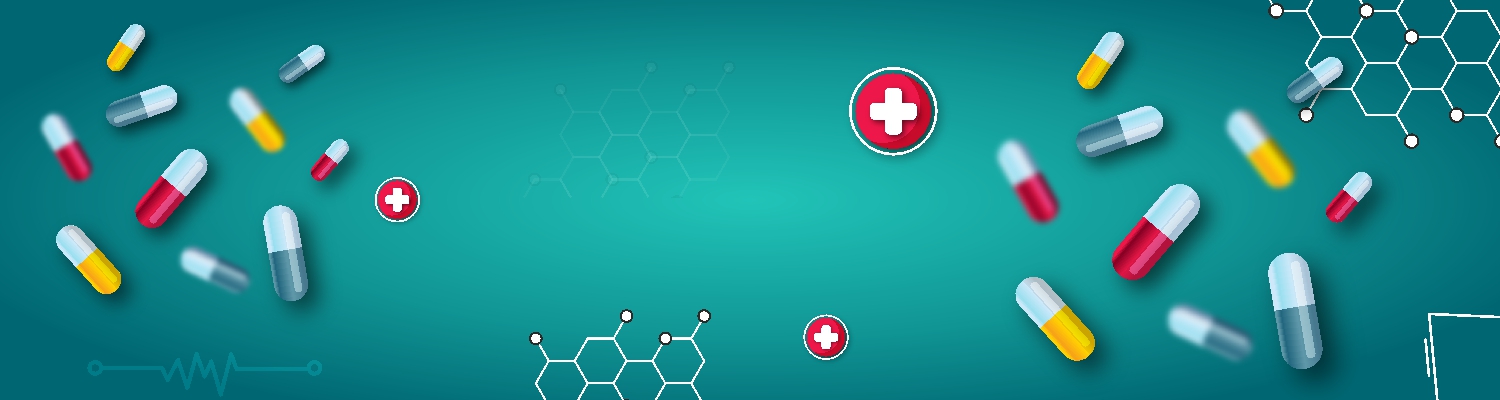
ISSN: 2637-6660
Farouk S Nas1, Muhammad Ali2* and Aminu Muhammad A2
Received: May 22, 2018; Published: May 31, 2018
*Corresponding author: Muhammad Ali, Microbiology Department, Kano University of Science and Technology Wudil Kano, Nigeria
DOI: 10.32474/ANOAJ.2018.01.000114
Bacterial strains resistant to the antibiotics now in use have becomes serious public health problems that increases the need to develop new bactericidal materials. Consequently, there is a strong demand for developing novel strategies and new materials that can cope with these problems. The emergence of nanotechnology has created many new antimicrobial options. The small size of the nanoparticles is very suitable for carrying out antimicrobial biological operations. Metals such as silver, zinc, copper and iron nanoparticle types have shown tremendous potential as bactericidal and fungicidal elements, demonstrating their potential as efficient antibiotic reagents in wound care and related medical issues. These nanomaterials displayed antimicrobial activity against numerous pathogenic viral and bacterial species. Nanomaterials today are a promising platform for alternative measures to control bacterial infections as they offer prolonged antimicrobial activity with negligible toxicity, compared with small molecular antimicrobial agents that display short term activity and environmental toxicity. The antimicrobial nanoparticle physically destroys cell membranes of the organism which prevent development of drug-resistance microbes.
Keywords: Antimicrobial Agents, Bacteria, Nanoparticles, Nanotechnology, Dwarf, Nano, Ribosomes, Antibiotics, Dna Replication, Metal Oxide Nanoparticles
Bacterial infections are still a major cause of morbidity and mortality. The growing concern regarding multi-drug resistant bacterial strains and bio film associated infections calls for the development of additional bactericidal means. Consequently, attention has been especially devoted to new and emerging nanoparticle-based materials in the field of antimicrobial chemotherapy [1]. The use of nanotechnology in various sectors of therapeutics has revolutionized the field of medicine where nanoparticles of dimensions ranging between 1-100nm are designed and used for diagnostics, therapeutics and as biomedical tools for research [2]. Nanoparticles are nano-sized mol¬ecules (usually less than 100nm in diameter) with a large sur¬face area to volume ratio, which helps them to easily penetrate bacterial cells. Commonly used elements in nanomolecules in¬clude gold, silver, copper, zinc, nickel, titanium, magnesium, alu¬minium, silicon, iron, calcium, and yttrium [3,4].
Nanotechnology is the study of extremely small structures. The prefix “nano” is a Greek word which means “dwarf”. The word “nano” means very small or miniature size. Nanotechnology is the treatment of individual atoms, molecules, or compounds into structures to produce materials and devices with special properties. The nanomaterials level is the most advanced at present, both in scientific knowledge and in commercial applications. Nanotechnology is being applied extensively to provide targeted drug therapy, diagnostics, tissue regeneration, cell culture, biosensors and other tools in the field of molecular biology [5]. Various nanotechnology platforms like fullerenes, nanotubes, quantum dots, nanopores, dendrimers, liposomes, magnetic nanopores and radio controlled nanoparticles are being developed [5]. Nanomaterials may be strategically advantageous as active antibacterial groups since their surface area is exceedingly large relative to their size. Nanosized particles may provide high activity although only a small dose of the particles is used. Consequently, nanomaterials could serve as an alternative to antibiotics to control bacterial infections. The major groups of antibiotics, currently in use, generally affect three bacterial targets: cell wall synthesis, translational machinery, and DNA replication [6].
Unfortunately, bacterial resistance may develop against each one of these modes of action. Mechanisms of resistance include enzymes that modify or degrade the antibiotic such as 𝛽-lactamases and aminoglycosides, modification of cell components such as cell wall as seen in vancomycin resistance [6] and ribosomes in tetracyclines resistance, and finally efflux pumps that provide multidrug resistance against numerous antibiotics [6]. Since nanoparticles’ mode of action is mainly by direct contact with the bacterial cell wall, without the need to penetrate the cells, most of the resistance mechanisms seen with antibiotics are irrelevant. This raises the hope that nanoparticles would be less prone than antibiotics to promote resistant bacteria [7]. Physiochemical properties of nanomolecules antimicrobial agents are widely used in human and veterinary medicine, as well as in water treatment and in the food (preservative) and textile industries [8]. Nanomolecules can be used as an alternative to conventional antimicrobial agents and can also act as carriers for antibiotics and other drugs. Nanomolecules can be synthesized from poly¬mers, lipids, and metals [9,10]. The paper was aimed to review the chemical properties of nanoparticles, their modes of action and potential use in medicine with special reference to antimicrobial properties.
Nanoparticles possess unique physical, chemical, electronic, electrical, mechanical, magnetic, thermal, dielectric, optical, and biological properties. Metal oxide nanoparticles are of great in¬terest for use as potential antimicrobial agents because of their unique optical, electronic, and magnetic properties. The electro¬static interaction of nanoparticles with negativelycharged bac¬terial surfaces draws the particles to the bacteria and promotes their penetration into the membrane. A strongly positive zeta potential of a nanoparticle promotes nanoparticle interactions with cell membranes leading to membrane disruption, bacterial flocculation, and a reduction in viability. The generation of reac¬tive oxygen species is also a mechanism of nanoparticle anti¬bacterial activity [11].
Further mechanisms of action of nanoparticles as antimicro¬bial agents include disrupting deoxyribonucleic acid during the replication and cell division of microorganisms, compromising the bacterial membrane integrity via physical interactions with the microbial cell (the physical presence of a nanoparticle most likely disrupts cell membranes in a dose-dependent manner), and releasing toxic metal ions and possessing abrasive properties which bring about lysis of cells [8].
The mechanism of action of nanoparticles on cell membranes is recognized as non-specific and it is not known if polymixins has any involvement on this process. Polymixins antibiotics can interact on cell membranes breaking vital barriers of microorganisms [12]. There is no doubt that cell permeability is altered when in contact to nanoparticles. Experiments indicated the formation of a “hole” or “pore” in the living cell membranes as a possible mechanistic hypothesis [13]. Although the meaning of the term “hole” or “pore” still requires clarification, images of cell damage has given clear evidence of this effect. In more serious or extreme cases a literal hole in the bilayer membrane exists which promotes the complete loss of the plasma membrane [14].
It has been demonstrated that Cd2+, Zn2+, Ag+ ions can react in bacteria with different groups of proteins. The ability of Ag+ in forming sparingly soluble salts is also considered as one of its major mechanisms for attacking bacteria cells. For instance, cell respiration is inhibited when the chloride ions precipitate as silver chloride in the cytoplasm of the cells. The antimicrobial efficiency of silver nanoparticles is also well-known, especially against Gramnegative bacteria such as E. coli. Hence, the silver nanoparticles act as antimicrobial releasing silver ions and also by penetrating cells interfering in their metabolic systems [14]. Although silver has received much attention, Cd2+ and Zn2+ ions can also bind to sulfur-containing proteins of the cell membrane and interfere in cell permeability. There are good evidences that Ag+ ions can also damage DNA by inhibiting its replication. The concentrations required for bactericidal activity of silver nanoparticles are low (in the range 10-9 mol/l) [14].
There is strong evidence that the positive charge of nanoparticles is critical for antimicrobial activity since bacteria cell membrane is negatively charged. Although the clear mechanism is still under debate, it has been suggested that ions (e.g. silver) can affect membrane-bound respiratory enzymes as well as affect efflux bombs of ions that can result in cell death [15]. The contact of bacteria has also given insights about key enzymes that can be inactivated. In general, the cascade of events after nanoparticle contact with bacteria can start with possible oxidation of respiratory enzymes, and so help facilitate the production of ROS (Reactive Oxygen Species) and radical species that will eventually affect cell physiology and promote DNA degradation [16,17].
The oxygen is a powerful oxidant agent and although it corresponds to the best acceptor of electrons during respiration it can be lethal for some bacteria. Triplet oxygen (3O2) is the ground state of the oxygen molecule and can be extremely poisonous for cells, but singlet oxygen (O2) can also be deadly for bacteria. It is well known that the generation of singlet oxygen can lead to peroxidation of cellular constituents such as proteins and lipids [18]. Singlet oxygen is a potent reagent promoting spontaneous and undesirable oxidations inside the cell. During many processes, H2O2 is formed by the respiratory burst which consumes O2 with the production of free radicals. Hence, the production of free hydroxyl radicals is certainly the basis of hydrogen peroxide action and evidence exists for this reaction leading to oxidation of DNA, proteins and membrane lipids [18]. Bacteria affected by ROS lose the integrity of their membranes progressively, disabling them of adhering to the surfaces, to maintain an appropriate communication with other bacteria, or to express other functions with efficiency. Many theories for explaining the mechanisms of action of nanoparticles involve the liberation of ROS and inhibition of the cellular adhesion. Several microorganisms can fight back as a response to ROS by producing enzymes, such as superoxide dismutase. This strategy can be a successful way to neutralize oxidative stress. In addition, coping with extreme oxidative stress, bacteria can present two important systems: SoxRS (responding to superoxide) and the OxyR (responding to hydrogen peroxide). These systems can be effective ways to repair damaged cell components and regulate reducing conditions [12,15,19]. Finally, it is well documented that bacteria exposed to nanoparticles present oxidative stress related to ROS.
The antibacterial properties of a variety of nanostructures have been investigated. In understanding the antibacte¬rial properties of these nanostructures, it is important to recognize that while some metals, such as zinc, silver, and copper, exhibit antibacterial mechanisms in their bulk form, other materials, such as iron oxide, are not antibacterial in their bulk form but may exhibit antibacterial properties in nanoparticulate form [19]. The mechanism of this antibacterial activity varies from nanoparticle to nanoparticle. For all varieties of nanoparticles, the antibacterial mechanism is not fully understood. While some proposed mechanisms relate to the physical structure of the nanoparticles (membrane-damaging abrasiveness of the nanoparticle), others relate to the enhanced release of antibacterial metal ions from nanoparticle surfaces [19]. The specific surface area of a dose of nanoparticles increases as the particle size decreases, allowing for greater mate¬rial interaction with the surrounding environment. Thus, for inherently antibacterial materials, such as zinc and silver, increasing the surface to volume ratio enhances the antibacterial effect. A nanoparticle of an inherently antibacte¬rial material may, therefore, have multiple mechanisms of antibacterial activity, such as the release of antibacterial metal ions from the particle surface and the antibacterial physical properties of a nanoparticle related to cell wall penetration or membrane damage. Comparing results across a greater number of studies allows for the identification of nanoparticle parameters which are most relevant in designing the ideal antibacterial particle. Chemistry, particle size, particle shape, and zeta potential are among the most relevant variables affecting antibacterial activity [19].
Recent studies have revealed that nanomolecules have excel¬lent biocidal properties to eliminate Salmonella typhi and can eradicate cancer-promoting Cyanobacteria and algae (Microcystis aeruginosa) from the environment [20,21]. In the era of the emergence and spread of microorganisms that are multidrug resistant, the potential antimicrobial activity of gold and silver nanomolecules should be considered as a positive indication of the utility of nanoparticles as antimicrobial agents [22]. A recent report has noted the potential of silver nanomolecules against bacteria [23]. Metal oxide nanoparticles of zinc (ZnO), copper (CuO) and iron (Fe2O3) were studied for their antimicrobial ac¬tivities against both Gram-positive bacteria (Staphylococcus aureus and Bacillus subtilis) and Gram-negative bacteria (Pseudomonas aeruginosa and Escherichia coli). This study revealed that ZnO nanoparticles demonstrated greatest antimicrobial ac¬tivity against both Gram-positive and Gram-negative bacteria while iron oxide nanomolecules showed the least antimicro¬bial potential [24]. The antibacterial and antifungal activities of ZnO nanoparticles were reported recently by [25], who found that ZnO was effective against both food pathogens (E. coli and P. aeruginosa) (100μL) and Aspergillus niger (400μL) at varied concentrations. This study emphasized the utility of nanoparticles in the food packaging industry. The synthesis of nanoparticles from plant sources (green synthesis) has been an emerging field of nanoscience in the recent past.
Reduced viability of S. aureus and E. coli was observed when the bacteria were exposed to ZnO nanoparticles of decreasing size. However, a concentration of 5mM (407.04μg/mL) was necessary to reduce viability of either bacteria species at 24 hours even with 40 nm diameter particles, the smallest diameter ZnO nanoparticle tested [26]. The two bacteria species tested were affected approximately equally by the presence of nanoparticles. Irregularity in cell membranes of bacteria exposed to ZnO nanoparticles was also noted. An increased antimicrobial effect of ZnO nanoparticles on E. coli was observed at the 18-hour time point as the particle diameter was reduced from 2μm to 45nm to 12nm, and this was attributed to the enhanced effect of the greater surface area to volume ratios and mechanical damage caused to the cells due to increased abrasiveness of the smaller nanoparticles [27]. A study which investigated the antibacterial effects of zinc ions identified MIC of 1917μg/mL, 9μg/mL, and 39μg/mL at 48 hours for Pseudomonas aeruginosa, S. aureus, and Candida albicans, respectively [28,29] Compared to P. aeruginosa, S. aureus was found to be far more sensitive to lower concentrations of zinc ions.
The activity of nanomolecules prepared from Allium species such as ginger and garlic, with silver as the metal (AgNO3), showed antimicrobial activity against common bacterial pathogens such as E.coli, Proteus spp., Klebsiella spp., Staphylococcus spp., Enterobacter spp., Bacillus spp., and Pseudomonas spp [30-33]. In a study comparing the morphological features of Gram-positive (S. aureus) and Gram-negative (E. coli) bacteria exposed to the same concentration of silver ions (10μg/mL silver nitrate for 4–12 hours), both bacteria species exhibited condensation of DNA, cell membrane separation from the cell wall, and cell wall damage [34]. Silver nanoparticles with an average diameter of 21nm were shown to inhibit the growth of Gram-negative bacteria species (E. coli, Vibrio cholerae, Salmonella typhi, and P. aeruginosa) on agar plates with nanoparticle concentrations of 75μg/mL [35]. To determine the ability of silver nanoparticle con-centrations to reduce bacteria growth on agar plates, silver nanoparticles with an average diameter of 12nm were incorporated into agar plates at concentrations of 10–100μg/mL [36]. E. coli was added to the plates at population densities of 105 CFUs. After 24 hours of growth, CFU populations on 10μg/mL plates were 70% lower than on control plates which did not contain silver nanoparticles [37]. A very recent report demonstrated that selenium and silver nanomolecules are produced by bacteria isolated from coal mines, and that such nanoparticles have been observed to have antibacterial activity against bacteria including E. coli, Klebsiella spp., Pseudomonas spp., and S. aureus [38,39].
Conventional copper, like conventional silver, exhibits antibacterial properties. Copper nanoparticles added to agar plates reduced E. coli and B. subtilis survival by 90% at concentrations of 33 μg/mL and 28μg/mL respectively [40]. In another study that utilized a unique method of impregnating filter paper with nanoparticles and placing pieces of filter paper on inoculated agar plates, the MIC of copper nanoparticles (mean average diameter = 10 nm) was determined to be 140–280μg/mL for E. coli, depending on the strain tested, and 140μg/mL for S. aureus strains [41]. Although conventional iron oxide is not considered anti-bacterial, a few studies on its effect on bacteria have been conducted and inhibition of bacteria activity has been observed. For example, iron oxide nanoparticles in a chain-like structure with a length of 100–200nm were shown to reduce S. aureus viability as illustrated by a decrease in the ratio of live to dead cells [42]. At concentrations of 3 mg/mL, iron oxide nanoparticles were shown to reduce cell viability at 4, 12, and 24 hours compared to bacteria culture controls with¬out nanoparticles and compared to lower concentrations of nanoparticles. As another example, iron oxide nanoparticles in Staphylococcus epidermidis suspensions reduced cell numbers at 12, 24, and 48 hour time points in a dosedependent matter, according to optical density readings [43]. After 48 hours, a concentration of 2 mg/mL reduced cell populations by about 65% compared to control groups with no nanoparticles. High concentrations of nanoparticles (100μg/mL, 1mg/mL, and 2mg/ mL) also increased the number of dead cells observed during a live/ dead assay. Antibacterial activity was again attributed to increasing oxidative stress and bacteria membrane interference.
In spite of possible limitations, nanotechnology represents an innovative strategy to develop and test new pharmaceutical formulations based on metallic nanoparticles with efficacious antimicrobial properties. Silver nanoparticles are the most studied metal and have several potential applications in medicine and dentistry. Other metallic nanoparticles and metal oxides of zinc, copper and iron are presenting interesting features that may compensate drawbacks from other species. Characteristics of nanoparticles such as size and morphology are important for their antimicrobial activity. Therefore, pre-clinical and clinical trials are urgently needed for a better understanding of potentiality and limitations when using metallic nanoparticles and elucidate the mechanisms involved with the antimicrobial activity of these nanoparticles.
Bio chemistry
University of Texas Medical Branch, USADepartment of Criminal Justice
Liberty University, USADepartment of Psychiatry
University of Kentucky, USADepartment of Medicine
Gally International Biomedical Research & Consulting LLC, USADepartment of Urbanisation and Agricultural
Montreal university, USAOral & Maxillofacial Pathology
New York University, USAGastroenterology and Hepatology
University of Alabama, UKDepartment of Medicine
Universities of Bradford, UKOncology
Circulogene Theranostics, EnglandRadiation Chemistry
National University of Mexico, USAAnalytical Chemistry
Wentworth Institute of Technology, USAMinimally Invasive Surgery
Mercer University school of Medicine, USAPediatric Dentistry
University of Athens , GreeceThe annual scholar awards from Lupine Publishers honor a selected number Read More...